Abstract
The biotechnological potential of Nostoc linckia as a biofertilizer and source of bioactive compounds makes it important to study its growth physiology and productivity. Since nitrogen is a fundamental component of N. linckia biomass, we compared the growth and biochemical composition of cultures grown in BG11 (i.e., in the presence of nitrate) and BG110 (in the absence of nitrate). Cultures grown in BG11 accumulated more cell biomass reaching a dry weight of 1.65 ± 0.06 g L–1, compared to 0.92 ± 0.01 g L–1 in BG110 after 240 h of culture. Biomass productivity was higher in culture grown in BG11 medium (average 317 ± 38 mg L–1 day–1) compared to that attained in BG110 (average 262 ± 37 mg L–1 day–1). The chlorophyll content of cells grown in BG11 increased continuously up to (39.0 ± 1.3 mg L–1), while in BG110 it increased much more slowly (13.6 ± 0.8 mg L–1). Biomass grown in BG11 had higher protein and phycobilin contents. However, despite the differences in biochemical composition and pigment concentration, between BG11 and BG110 cultures, both their net photosynthetic rates and maximum quantum yields of the photosystem II resulted in similar.
Graphical Abstract

Similar content being viewed by others
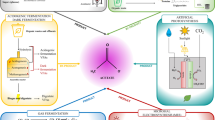
Avoid common mistakes on your manuscript.
1 Introduction
Cyanobacteria have been proposed as cell factories to produce high-value products such as carbohydrates, proteins, and lipids [1], food supplements and biomaterials [2, 3], as well as to produce biogas, and hydrogen [4,5,6,7].
Cyanobacteria perform oxygen-evolving photosynthesis, split water and synthesize organic molecules using solar energy. In contrast to microalgae and higher plants the plastoquinone pool is shared between the photosynthesis chain and respiration. This fact can affect the measure of fluorescence since respiration can reduce plastoquinone pool and promote state two transition. Another fundamental difference is that cyanobacteria possess only chlorophyll a, which is mainly associated with photosystem I (PSI). In addition, in cyanobacteria the content of PSI is up to five times higher than that of photosystem II (PSII). Phycobilisomes, accessory pigments, serving PSII help to balance light absorption between the two photosystems.
In recent years, the potential of cyanobacteria to produce plant biostimulants is being investigated [8, 9]. Cyanobacterial strains can be selected to produce compounds such as auxins and cytokinins, as well as biopesticides [10,11,12]. Several cyanobacterial species have shown remarkable pharmacological and biological properties for their ability to synthesize useful bioactive compounds [13, 14].
The use of cyanobacterial species offers many advantages, such as a rapid growth rate, high solar energy conversion efficiency compared to land plants, good compatibility with various pollutants, and the possibility of using wastewater sources for their growth [12, 15]. Their growth depends on culture conditions such as light, temperature, pH, and nutrient availability [16, 17]. Among nutrients, nitrogen (N) along with phosphorus (P) play an important role in the growth and photosynthetic performance [18,19,20]. N is essential for protein synthesis, and low N can reduce cellular growth rates and cause the degradation of phycobiliproteins, leading to the inhibition of photosynthesis [21, 22]. P plays both structural (i.e., for nucleic acids and cell membranes) and functional roles in a number of metabolic processes. Low P concentrations affect photosynthesis, ATP-dependent enzyme activity, and respiration [23].
The Nostocaceae is a family of cyanobacteria that form filamentous colonies and can fix atmospheric nitrogen in the absence of combined nitrogen. The filaments are embedded in a protective gelatinous matrix and consist of photosynthetic cells and N2- fixing heterocysts [24]. The development of a heterocyst occurs through the differentiation of a vegetative cell. During the development of heterocyst, the PSII is lost and there are fewer phycobiliproteins. By means of PSI, the heterocyst captures light to generate chemical energy. The absence of the PSII in the heterocyst prevents the release of O2 which would inactivate the nitrogenase, but it also means that neither electron nor reducing power are generated from water splitting operated by PSII. As a result, the heterocyst depends on nearby vegetative cells for the supply of reducing equivalents, which are likely imported in the form of carbohydrates. The enzyme nitrogenase catalyzes nitrogen fixation, where ammonia synthesis is accompanied by the generation of H2.
However, the presence of heterocysts is not necessarily proof that the organism is fixing N2. When cultures of heterocystous cyanobacteria are grown in the presence of combined nitrogen, heterocysts do not always disappear [25].
There is a growing interest in the cultivation of Nostoc species due to their wide range of applications [8, 26]. Bioactive chemicals of Nostocaceae species include the polysaccharide k-carrageenan (kcar), auxins, phycocyanin, and antibiotic-like compounds [8, 27]. Nostoc species have various antioxidant properties due to their ability to scavenge free radicals and inhibit lipid peroxidation [28]. Mai et al. [29] showed in their work that inoculation of Nostoc calcicola HN9 extract reduced the formation of O2− and H2O2 in leaves of soybean (Glycine max (L.)) infested with Aphis craccivora. Takács et al. [30] demonstrated the plant biostimulator effect of Nostoc piscinale on the winter wheat variety Bőség. Yadav et al. [31] showed that the culture extract of locally isolated Nostoc calcicola exhibited antibacterial activity against human pathogenic bacteria Escherichia coli and Staphylococcus aureus. Biondi et al. [32] reported that the Nostoc strain ATCC 53789 acts as a natural pesticide against a variety of plant-pathogenic fungi. Virág et al. [33] showed that freeze-dried and ultrasonically treated Nostoc biomass can promote the development of some orchid species. Nostoc calcicola is a rich source of pigments such as phycocyanin, allophycocyanin, phycoerythrin, and carotenoids that have medicinal, industrial, and biotechnological applications [34]. It is also a good candidate for the decontamination of cadmium by microbially induced carbonate precipitation [35].
The objective of this study was to investigate whether there are significant differences in the productivity and biochemical composition of the biomass of the cyanobacterium Nostoc linckia (N. linckia) grown in two different culture media. To this end, N. linckia was grown in BG11 (i.e., with nitrate) and diazotrophically in BG110 (i.e., without nitrate) under fixed conditions of light/dark cycle, light intensity, and temperature. The results are important to properly address the choice of the culture medium in large-scale cultures, where the nitrogen supply can represent an important voice of the production cost of this cyanobacterium to produce phyto-stimulants, biofertilizers and valuable pigments such as phycobilin.
2 Material and methods
2.1 Culture conditions
The freshwater filamentous cyanobacterium N. linckia strain MACC-612 (previously referred to as Nostoc calcicola strain MACC-612) (Fig. 1) was obtained from the Mosonmagyaróvár Algal Culture Collection (Hungary). Growth experiments were performed in vertical glass columns (5-cm light path, 400 mL working volume). N. linckia cells were grown in BG11 and BG110 (BG11 without nitrate) media in batch mode under specified conditions of light/dark cycle (10 h light/14 h dark), light irradiation (150 μmol photons m–2 s–1), temperature (28 °C) and mixing (mixture of air/CO2 98:2 v/v). Light irradiation was provided by cool white lamps (Dulux L, 55 W/840, Osram, Italy). The starting biomass dry weight of both culture conditions was 44 mg L–1. The experiments lasted for ten days. Composition of the BG11 medium [36]: (g L–1): NaNO3 1.5, CaCl2*2H2O 0.036, MgSO4*7H2O 0.075, K2HPO4 0.04, Citric Acid 0.006, (NH4)5[Fe(C6H4O7)2] 0.006, Na2CO3 0.02, Na2-EDTA 0.001, H3BO3 0.0028, ZnSO4*7H2O 0.00022, MnCl2*4H2O 0.0018, NaMoO4*2H2O 0.00039, CuSO4*5H2O 0.00008, Co(NO3)2*6H2O 0.00005, pH7.2. Photon flux density (PFD) was measured using the LI-250A equipped with a quantum radio-photometer (LI-COR Biosciences). NaNO3 was not added to the BG110 medium.
At the beginning and end of each light cycle, samples were taken from the cultures to determine phosphorus and nitrogen consumption, cell growth (as CDW), and photosynthetic activity (oxygen evolution and respiration rate and chlorophyll fluorescence). Sample volume was 30 mL. and thereafter once the cultures were denser, we reduced the volume withdrawal from the tubes to 20 mL and then to 10 mL.
2.2 Analytical procedures
Cell biomass dry weight (CDW) (g L–1) was measured in triplicate using 5 mL culture samples at the beginning and end of the light period using the method described by Touloupakis et al. [37]. Culture samples are filtered using pre-weighed 47 mm diameter glass microfiber filter membranes (Whatman GF/F, Maidstone, England), washed twice with deionized water, dried at 80 °C until constant weight. Chlorophyll content was measured spectrophotometrically in triplicate with 5 mL samples centrifuged in an ALC-PK110 centrifuge at 2650g for 8 min. The pellet was resuspended in 5 mL of pure methanol, placed in a 70 °C water bath for 3 min, and centrifuged again. The absorbance of the supernatant was measured at 665 and 750 nm [38]. Extraction of phycobiliproteins from fresh biomass performed according to the method described by Lauceri et al. [39]. 5 mL of the culture was centrifuged at 12,000g for 10 min (T = 10 °C). The pellet was washed once with pure Milli-Q water and centrifuged again for 10 min. The pellet was suspended in 10 mL of extraction solution, freeze-thawed, and sonicated.
During the experiments, samples of the culture were collected (5 mL), at the beginning and end of the light period, and analyzed for nitrate concentration (NO3–) using a DX-320 ion chromatograph (Dionex, Sunnyvale, CA, USA) equipped with a conductivity detector and an AD25 Ultraviolet–Visible detector. Separations were performed using a DionexIonPac AS19 (250 × 4.0 mm) analytical column and an IonPac AG19 (50 × 4.0 mm) guard column. The AQuAAtro segmented flow analyzer (Seal Analytical, Norderstedt, Germany) was used for phosphate (PO4) analysis.
Chlorophyll fluorescence measurements were performed using a pulse-amplitude-modulation fluorometer (PAM-2100, H. Walz, Effeltrich, Germany) operated by PamWin PC software. The Fv/Fm [(Fm – F0)/Fm] ratio between variable and maximum fluorescence, was used to determine the maximum photochemical quantum yield of PSII in dark-adapted culture samples. Where F0 is the minimal fluorescence level with all PSII reaction centers open, and Fm is the maximum fluorescence yield. For this purpose, samples were incubated in the dark for 5 min to remove any energy-dependent quenching. In addition, just before sending a flash for the Fm determination, the sample was illuminated with a 10 s-long far-red light pulse (above 700 nm, 10 W m−2), supplied by the PAM-2100.
The nonlinear least squares regression model of Eilers and Peeters [40] was used to fit electron transport rate/PFD curves obtained from the culture samples. Each curve allowed the determination of the following photosynthetic variables: irradiance at the onset of light saturation (Ik), and light utilization efficiency as determined by the initial slope of P/I curve (α). The maximum electron transport rate (ETRmax) was calculated as the product of the effective photochemical quantum yield of PSII and the photon flux density. Oxygen evolution measurements were performed in triplicate with 2 mL culture samples using a liquid-phase oxygen electrode chamber (DW3 Hansatech, UK) equipped with an oxygen control electrode unit (Oxy-lab Hansatech, UK). Light was supplied by a red LED light source (LH36/2R Hansatech, UK) with a wavelength of 637 nm and 500 μmol photons m−2 s−1 PFD [41].
Electron transport rates were calculated as ETR = Y(II) × PAR × ETR factor, where ETR is the electron transport rate, Y(II) is the effective quantum yield, PAR is the incident irradiance based on the photosynthetically active radiation (PAR) list of the instrument (in µmol photons m–2 s–1) and ETR factor is the fraction of light absorbed by the sample and distributed to PSII (for ETR calculations, ETR factor of 0.42 was used arbitrarily, since true PSII absorption requires quantitative information on the PSII absorption cross section) [42].
2.3 Statistical analysis
Data were analyzed using one-way analysis of variance (ANOVA) at a significance level of p < 0.05, after the homogeneity test. Statistical analysis was performed using GraphPad Prism version 6.00 (GraphPad Software, California, USA).
3 Results and discussion
The availability of nutrients has a significant impact on the growth of cyanobacterial cultures. To produce biomass for biotechnological purposes, nitrogen content in the growth medium is a crucial parameter. Protein and carbohydrate contents can be affected by the availability of growth medium components such as nitrate and phosphate. Nitrogen is a vital ingredient that influences cell development and physiology, and its scarcity can have a variety of consequences for cyanobacteria metabolism.
Considering that cyanobacteria are currently produced commercially under natural conditions, it is critical to understand the effects of irradiance and photoperiod on the photosynthetic activity, biomass production and composition. The composition of the growth medium can affect photosynthesis, respiration, growth rate, and cellular components such as pigments, carbohydrates, and proteins [43]. In this work, N. linckia cells were cultured for ten days in BG11 and BG110 growth media under fixed light/dark cycle conditions. In this study, cells were cultured under a 10/14 L/D to mimic the natural light-dark cycle. Dron et al. demonstrated that the photosynthetic machinery on the thylakoid membrane is altered in response to cell cycling, which could alter photosynthesis [44]. The photosynthetic rate in microalgal cells synchronized by L/D phases can vary by more than twofold [45]. During the cell cycle, there can be significant changes in the structure of thylakoid membranes, in addition to changes in proteins for light collection and electron transport [46].
The macronutrient composition of the growth media influenced N. linckia cell growth. The cells formed aggregates after the first day of growth in BG110, while in BG11-grown cells, no cell aggregation was observed. In the absence of air bubbles, the cells in BG110 sedimented immediately, while the cells in BG11 remained in suspension for a long time (Fig. 2). It is known that cyanobacteria increase exopolysaccharide production under conditions of nitrogen deficiency [47]. The ability of cyanobacterial polysaccharides to protect nitrogenase from the negative effects of oxygen is one possible function. Prosperi showed that the thick mucilaginous shell of heterocysts of a strain of Nostoc cordubensis appears to be critical in preventing the inactivation of nitrogenase activity by atmospheric oxygen [48].
In both culture media, CDW increased constantly under light conditions, whereas it decreased in the dark due to carbohydrate metabolism which caused loss of dry weight. Cultures in BG11 achieved a higher CDW (1.65 ± 0.06 g L–1) compared with BG110 (0.92 ± 0.01 g L–1) (Fig. 3A). Biomass productivity was higher in the culture with BG11 medium (average 317 ± 38 mg L–1 day–1), in the last five days, compared to that with BG110 medium (average 262 ± 37 mg L–1 day–1) in the last three days. N. linckia cells cultured in BG11 showed a much higher chlorophyll concentration (39.0 ± 1.3 mg L–1) than in BG110 (13.6 ± 0.8 mg L–1) (Fig. 3B).
Heterocyst in cyanobacteria can fix atmospheric nitrogen to produce chlorophyll and carotenoids. However, nitrogen fixation comes at a high energy cost, requiring 16 ATP and eight electrons per fixed N2 [49]. Cyanobacteria do not produce active heterocysts in conditions where available N, such as ammonium and nitrate, is a non-limiting resource [50, 51]. Many cellular systems are strained by such a substantial investment in N2 reduction, resulting in lower growth rates [52]. Despite the BG11 culture’s phosphate depletion on day 8, the growth was still remarkable probably sustained by increased synthesis of carbohydrate which were then partially metabolized at night to sustain respiration (Fig. 3).
It is known that nitrogen absence in the culture medium stimulates polysaccharide production as it has been demonstrated in Nostoc [53], Oscillatoria formosa [54], and Anabaena [55]. The presence of exopolysaccharides coats on their trichomes facilitates colony formation and enabling them to retain moisture for growth and protect cells from various environmental stresses [56]. Data collected in BG110 cultures showed that pigment production is possible in the absence of nitrate and that the amount of chlorophyll correlates with cell biomass, confirming the good diazotrophic capacity for growth and pigment production in this strain [57] (Table 1). Accessory pigments such as phycocyanin, phycoerythrin and allophycocyanin concentrations were predominant in BG11. Protein content was higher in BG11 cultures, while carbohydrates were higher in BG110 (Table 1). Therefore, the metabolism of N. linckia cultures grown under diazotrophic conditions is more addressed toward the production of carbohydrate as it is usually observed in cyanobacteria grown under the limitation of nitrogen [58].
Khajepour et al. [43] investigated the influence of photoperiod and light intensity on N. calcicola growth and biochemical composition. Their results indicated that by increasing light intensity chlorophyll a, phycocyanin, allophycocyanin, and phycoerythrin content decreased while total carotenoids increased.
Differences in growth depending on environmental factors such as temperature, light, and pH, can even be found within strains of the same cyanobacterial species [59]. N. linckia does not require a nitrogen source in the culture medium because its growth is sustained by the process of nitrogen fixation although at a lower rate compared to BG11.
Figure 4 shows the change in nitrate and phosphate content of each culture during the experiment. The initial phosphate content in both growth media was 23 mg L–1, while the average nitrate content in BG11 cultures at the start of the experiments was 1.1 g L–1. In BG11, nitrate gradually decreased, and at the end of the experiment a concentration of 864 mg L–1 was detected (Fig. 4). The kinetics of phosphate consumption was faster in BG11 cultures very likely because of faster growth. In this culture, the phosphate concentration was completely depleted after 192 h, while in BG110 there was a residue 1.85 mg L–1 at the end of the experiment.
Table 1 shows the pigment and biochemical composition of N. linckia cultures grown in BG11 and BG110. Cells grown in BG11 showed increased pigment and protein content, indicating that the presence of available nitrogen strongly influences the composition of cells. Chlorophyll content was 40% higher in cell grown with nitrate. Valuable pigments such as phycocyanin, phycoerythrin, allophycocyanin were significantly higher (p < 0.01) in cells grown in the presence of nitrate (BG11).
Chlorophyll a is the primary pigment involved in photosynthetic activity in PSI and PSII, although carotenoids are critical for light absorption and protection from light-induced oxidation [60]. Therefore, higher carotenoid content is considered an indication of protection against reactive oxygen species (ROS) which may cause photodamage of PSII. Lower chlorophyll content usually indicates that culture was tendentially acclimated to high light. Indeed, the cell density in the BG110 culture was lower than in the BG11 culture. Therefore, specific light absorption by BG110 cells was higher. However, the fluorescence and oxygen evolution results indicated that no photoinhibition occurred under either culture condition, indicating that the culture was effectively protected from photoinhibition, both by carotenoids and most likely by an effective repairing cycle of PSII [61, 62].
Protein content in cells grown diazotrophycally was much lower than in cells grown in the presence of nitrate (36.8% vs 55.5%).
Net oxygen evolution rate measurements of N. linckia cells were carried out at the beginning and end of each light/dark period of the cultures (Fig. 5). In BG11 cultures, oxygen evolution increased during the light period until day 6 and then remained stable. The maximum oxygen evolution rate (960 µmol O2 mg chl–1 h–1) was observed after 96 h when the chlorophyll concentration was 14.4 ± 0.6 mg L–1. This value is close to the value (935 µmol O2 mg chl–1 h–1) found in the work of Celis-Pla and coworkers using the same Nostoc strain in outdoor cultures [8]. In BG11 culture, the net oxygen evolution activity during the first 5 days (120 h) was higher than that measured in BG110 cells. Thereafter, it showed a remarkable reduction stabilizing around a value 50% lower than the maximum attained. Reduction in photosynthetic activity was also accompanied by a reduction in the respiration rate (Fig. 5B). This condition coincided with a strong drop in the phosphate availability in the culture medium. Therefore, it is conceivable that the reduction in the photosynthetic performance may be attributed to a phosphate limitation [23]. The general pattern of photosynthesis in both cultures was bell-shaped, with a maximum of 120–140 h, after acclimation to low light due to the increased density of the cultures. Dark respiration rates on chlorophyll basis increased immediately in both growth media to a kind of stationary phase in the range of 335–470 μmol O2 mg chl–1 h–1 and 100–224 μmol O2 mg chl–1 h–1 for BG110 and BG11, respectively. Respiration then began to decline on day 5 in BG11 and day 6 in BG110 (Fig. 5B).
Changes in net oxygen evolution (A) and respiration rates (B) with a time of N. linckia cells cultivated in BG11 (white circles) and BG110 (black circles) growth media. The white and grey columns represent the light and dark periods, respectively. The error bars represent a standard deviation from the average of three specimens
It is noteworthy that gross photosynthesis rates, the sum of the net rate plus the respiration rate, were considerably higher in cells grown diazotrophycally. This fact may result in contrast with the lower growth attained by cultures grown in BG110 (Fig. 3). This apparent contrast may be explained by the fact that cells grown diazotrophycally must drain a considerable amount of ATP and NADPH2 produced by the photosynthesis to meet the high energetic requests by nitrogen fixation [63]. Acclimation to low light induces an increase in chlorophyll and a decrease in the saturation of photosynthesis [64]. The increase in photosynthesis in BG110 cells is explained by the fact that the culture in BG110 becomes acclimated to high light intensity, characterized by a reduction in chlorophyll per dry weight. In fact, chlorophyll/DW was 0.75% in BG110, and 1.28% in BG11. Cells acclimated to high light are characterized by a higher photosynthesis rate per unit of chlorophyll a [64,65,66]. This behavior was initially noticed in Nannochloropsis cells during the transition from bright light to dim light; they discovered that acclimation to dim light increased photosynthetic unit turnover time [67]. Similar behavior was also observed with Chlamydopodium fusiforme cultures grown in media with different nitrogen concentrations [68].
Analysis of chlorophyll fluorescence provides a noninvasive, fast evaluation of photosynthetic electron transport and light capture processes. Therefore, information on both photosynthesis and the acclimation status of cyanobacteria can be obtained rapidly from the chlorophyll fluorescence signals. The maximum quantum yield of PSII (Fv/Fm) values, in both culture media, showed only little fluctuations, and however within a physiologically range (0.40–0.55) (Fig. 6). It is well known that in cyanobacteria the value of Fv/Fm is usually lower than that found in microalgae, due to the influence of the respiration which reduces the plastoquinone pool (PQ pool) promoting state 1 to state 2 transition of photosynthetic apparatus. Indeed, in cyanobacteria, PQ pool is shared by both photosynthesis and respiration [69].
Changes in maximum quantum yield of PSII photochemistry (Fv/Fm) of N. linckia cells grown in BG11 (white circles) and BG110 (black circles). The white and grey columns represent the light and dark periods, respectively. The error bars represent a standard deviation from the average of three specimens
When comparing ETRmax values during the stationary phase in BG110 (48.0–56.8 μmol e– m–2 s–1) and in BG11 (45.1–62.0 μmol e– m–2 s–1), we observe only a slight difference (10%). There was no consistent change in initial slope (α) between the two culture conditions (Fig. 7B). In both culture conditions, an increase in ETRmax was observed (first 5 days) followed by a stationary phase (Fig. 7A). This behavior can be explained by the acclimation process of the cell to the light during the first 5 days of cultivation. Later, with the increase in the self-shading, cells started to acclimate to low light and ETR stabilized. Interestingly, comparing the ETR behavior to that of the photosynthesis we can observe that there was a good correlation between the two parameters mainly during the first 120 h of culture, thereafter while the ETR stabilized while the photosynthesis rate decreased. However, comparison of these two parameters in cyanobacteria is quite difficult since these organisms during acclimation to light can go through important changes in the composition of pigments, which can affect their optical cross section, and changes in the ratio between PSI/PSII, which can reach a value of up to 5 which affects the fluorescence yield [70, 71].
Nitrogen limitation can lead to a reduction in pigmentation and a reduction in the cellular concentration of reaction centers [72, 73]. This was verified in the BG110 culture, which had low carotenoid content (average of 0.30 ± 0.03 mg L–1) due to nitrogen depletion. N. linckia cells cultured in BG11 showed an increasing trend of carotenoid content with a maximum of 2.08 mg L–1 on day 7 (168 h) (Fig. 8). It then decreased sharply on day 9 very likely because of the exhaustion of phosphate in the medium (Fig. 4).
4 Conclusions
The amount of nitrogen contained in the culture media clearly affected the growth of N. linckia cells. Direct supply of nitrogen promoted better growth and enhanced the protein content as well as the production of valuable pigments such as phycocyanin, phycoerythrin and allophycocyanin with high market value. On the contrary, diazotrophic growth was mostly addressed to the production of carbohydrates. The different biochemical composition of the biomass strongly influences its commercial value. No effects on photosynthetic performance were observed in either culture condition. A biomass rich in nitrogen is particularly appreciated for use as an organic fertilizer, while a biomass rich in carbohydrates (polysaccharides) is appreciated for its capacity to improve soil fertility by improving soil structure [74]. Another very important biotechnological difference observed between the cultures was the rapid cell sedimentation observed in the culture grown in BG110. This is a very important issue since it may facilitate biomass harvesting from open cultivation systems [75]. Yet, rapid sedimentation can entail a high energy requirement for mixing to maintain good exposure of the cell to light. According to Norsken et al. [76], mixing accounts for about 26% of the cost of production. The phenotypic plasticity of this organism allows acclimation to different nutrient conditions by modulating its biomass composition. This makes it very attractive for biomass production for various purposes, such as aquaculture by cultivation in wastewater with high nitrogen content (e.g., digestate), for the production of nitrogen-rich biofertilizers, and for the extraction of bioactive substances and pigments.
Data availability
The data that support the findings of this study are available from the corresponding author upon reasonable request.
References
Liu, D., Liberton, M., Hendry, J. I., Aminian-Dehkordi, J., Maranas, C. D., & Pakrasi, H. B. (2021). Engineering biology approaches for food and nutrient production by cyanobacteria. Current Opinion in Biotechnology, 67, 1–6. https://doi.org/10.1016/j.copbio.2020.09.011
Hu, J., Nagarajan, D., Zhang, Q., Chang, J.-S., & Lee, D.-J. (2018). Heterotrophic cultivation of microalgae for pigment production: A review. Biotechnology Advances, 36(1), 54–67. https://doi.org/10.1016/j.biotechadv.2017.09.009
Sathasivam, R., Radhakrishnan, R., Hashem, A., & Abd Allah, E. F. (2019). Microalgae metabolites: A rich source for food and medicine. Saudi Journal of Biological Sciences, 26(4), 709–722. https://doi.org/10.1016/j.sjbs.2017.11.003
Seibert, M., & Torzillo, G. (2017). Microalgal hydrogen production: Achievements and perspectives. Royal Society of Chemistry, UK. https://doi.org/10.1039/9781849737128
Chew, K. W., Yap, J. Y., Show, P. L., Suan, N. H., Juan, J. C., Ling, T. C., Lee, D.-J., & Chang, J.-S. (2017). Microalgae biorefinery: High value products perspectives. Bioresource Technology, 229, 53–62. https://doi.org/10.1016/j.biortech.2017.01.006
Singh, H., & Das, D. (2018). Biofuels from Microalgae: Biohydrogen. In E. Jacob-Lopes, L. Queiroz Zepka, & M. I. Queiroz (Eds.), Energy from Microalgae (pp. 201–228). Springer. https://doi.org/10.1007/978-3-319-69093-3_10
Lindblad, P., Fuente, D., Borbe, F., Cicchi, B., Conejero, J. A., Couto, N., Čelešnik, H., Diano, M. M., Dolinar, M., Esposito, S., Evans, C., Ferreira, E. A., Keller, J., Khanna, N., Kind, G., Landels, A., Lemus, L., Noirel, J., Ocklenburg, S., … Wünschiers, R. (2019). CyanoFactory, a European consortium to develop technologies needed to advance cyanobacteria as chassis for production of chemicals and fuels. Algal Research, 41, 101510. https://doi.org/10.1016/j.algal.2019.101510T
Celis-Plá, P. S. M., Agustín Rearte, T., Neori, A., Masojídek, J., Bonomi-Barufi, J., Álvarez-Gómez, F., Ranglová, K., Carmo da Silva, J., Abdala, R., Gómez, C., Caporgno, M., Torzillo, G., Margarita Silva Benavides, M. A., Ralph, P. J., Fávero Massocato, T., Atzmüller, R., Vega, J., Chávez, P., & Figueroa, F. L. (2021). A new approach for cultivating the cyanobacterium Nostoc calcicola (MACC-612) to produce biomass and bioactive compounds using a thin-layer raceway pond. Algal Research, 59, 102421. https://doi.org/10.1016/j.algal.2021.102421
Santini, G., Biondi, N., Rodolfi, L., & Tredici, M. R. (2021). Plant biostimulants from cyanobacteria: An emerging strategy to improve yields and sustainability in agriculture. Plants, 10, 643. https://doi.org/10.3390/plants10040643
Tan, C. Y., Dodd, I. C., Chen, J. E., Phang, S.-M., Chin, C. F., Yow, Y.-Y., & Ratnayeke, S. (2021). Regulation of algal and cyanobacterial auxin production, physiology, and application in agriculture: An overview. Journal of Applied Phycology, 33, 2995–3023. https://doi.org/10.1007/s10811-021-02475-3
Chojnacka, K., Michalak, I., Dmytryk, A., Gramza, M., Słowiński, A., & Górecki, H. (2015). Algal extracts as plant growth biostimulants. In S.-K. Kim & K. Chojnacka (Eds.), Marine algae extracts: Processes, products, and applications (pp. 189–212). Wiley. https://doi.org/10.1002/9783527679577.ch11
Singh, J. S., Kumar, A., Rai, A. N., & Singh, D. P. (2016). Cyanobacteria: A precious bio-resource in agriculture, ecosystem, and environmental sustainability. Frontiers in Microbiology, 7, 529. https://doi.org/10.3389/fmicb.2016.00529
Singh, M., Singh, R., Parihar, P., Singh, M., Bajguz, A., Kumar, J., Singh, S., Singh, V. P., & Prasad, S. M. (2017). Uncovering potential applications of cyanobacteria and algal metabolites in biology, agriculture and medicine: Current status and future prospects. Frontiers in Microbiology, 8, 515. https://doi.org/10.3389/fmicb.2017.00515
Nandagopal, P., Steven, A. N., Chan, L. W., Rahmat, Z., Jamaluddin, H., & Mohd Noh, N. I. (2021). Bioactive metabolites produced by cyanobacteria for growth adaptation and their pharmacological properties. Biology (Basel), 10(10), 1061. https://doi.org/10.3390/biology10101061
Khalifa, S. A. M., Shedid, E. S., Saied, E. M., Jassbi, A. R., Jamebozorgi, F. H., Rateb, M. E., Du, M., Abdel-Daim, M. M., Kai, G.-Y., Al-Hammady, M. A. M., Xiao, J., Guo, Z., & El-Seedi, H. R. (2021). Cyanobacteria—from the oceans to the potential biotechnological and biomedical applications. Marine Drugs, 19, 241. https://doi.org/10.3390/md19050241
Lee, E., Jalalizadeh, M., & Zhang, Q. (2015). Growth kinetic models for microalgae cultivation: A review. Algal Research, 12, 497–512. https://doi.org/10.1016/j.algal.2015.10.004
Crnkovic, C. M., May, D. S., & Orjala, J. (2018). The impact of culture conditions on growth and metabolomic profiles of freshwater cyanobacteria. Journal of Applied Phycology, 30(1), 375–384. https://doi.org/10.1007/s10811-017-1275-3
Xin, L., Hong-Ying, H., Ke, G., & Ying-Xue, S. (2010). Effects of different nitrogen and phosphorus concentrations on the growth, nutrient uptake, and lipid accumulation of a freshwater microalga Scenedesmus sp. Bioresource Technology, 101, 5494–5500. https://doi.org/10.1016/j.biortech.2010.02.016
Jiang, Y., Yoshida, T., & Quigg, A. (2012). Photosynthetic performance, lipid production and biomass composition in response to nitrogen limitation in marine microalgae. Plant Physiology and Biochemistry, 54, 70–77. https://doi.org/10.1016/j.plaphy.2012.02.012
Kramer, B. J., Jankowiak, J. G., Nanjappa, D., Harke, M. J., & Gobler, C. J. (2022). Nitrogen and phosphorus significantly alter growth, nitrogen fixation, anatoxin-a content, and the transcriptome of the bloom-forming cyanobacterium, Dolichospermum. Frontiers in Microbiology, 13, 955032. https://doi.org/10.3389/fmicb.2022.955032
Richaud, C., Zabulon, G., Joder, A., Thomas, J., & Zabulon, R. (2001). Nitrogen or sulfur starvation differentially affects phycobilisome degradation and expression of the nblA gene in Synechocystis strain PCC 6803. Journal of Bacterioliology, 183, 2989–2994. https://doi.org/10.1128/JB.183.10.2989
Klotz, A., Reinhold, E., Doello, S., & Forchhammer, K. (2015). Nitrogen starvation acclimation in Synechococcus elongatus: Redox-control and the role of nitrate reduction as an electron sink. Life, 5, 888–904. https://doi.org/10.3390/life5010888
Tripathi, K., Sharma, N. K., Kageyama, H., Takabe, T., & Rai, A. K. (2013). Physiological, biochemical and molecular responses of the halophilic cyanobacterium Aphanothece halophytica to Pi-deficiency. European Journal of Phycology, 48, 461–473. https://doi.org/10.1080/09670262.2013.859303
Sand-Jensen, K. (2014). Ecophysiology of gelatinous Nostoc colonies: Unprecedented slow growth and survival in resource-poor and harsh environments. Annals of Botany, 114(1), 17–33. https://doi.org/10.1093/aob/mcu085
Stal, L. J. (2015). Nitrogen fixation in cyanobacteria. In eLS, John Wiley & Sons, Ltd (Ed.). https://doi.org/10.1002/9780470015902.a0021159.pub2
Ördög, V., Stirk, W. A., Takács, G., Pőthe, P., Illés, Á., Bojtor, C., Széles, A., Tóth, B., van Staden, J., & Nagy, J. (2021). Plant biostimulating effects of the cyanobacterium Nostoc piscinale on maize (Zea mays L.) in field experiments. South African Journal of Botany, 140, 153–160. https://doi.org/10.1016/j.sajb.2021.03.026
Niveshika, V. E., Mishra, A. K., Singh, A. K., & Singh, V. K. (2016). Structural elucidation and molecular docking of a novel antibiotic compound from cyanobacterium Nostoc sp. MGL001. Frontiers in Microbiology, 7, 1899. https://doi.org/10.3389/fmicb.2016.01899
Li, A. H., Cheng, K., Wong, C., King-Wai, F., Feng, C., & Yue, J. (2007). Evaluation of antioxidant capacity and total phenolic content of different fractions of selected microalgae. Food Chemistry, 102, 771–776. https://doi.org/10.1016/j.foodchem.2006.06.022
Mai, V. C., Nguyen, B. H., Nguyen, D. D., & Nguyen, L. A. (2017). Nostoc calcicola extract improved the antioxidative response of soybean to cowpea aphid. Botanical Studies, 58, 55. https://doi.org/10.1186/s40529-017-0211-9
Takács, G., Stirk, W. A., Gergely, I., Molnár, Z., van Staden, J., & Ördög, V. (2019). Biostimulating effects of the cyanobacterium Nostoc piscinale on winter wheat in field experiments. South African Journal of Botany, 126, 99–106. https://doi.org/10.1016/j.sajb.2019.06.033
Yadav, S., Agrawal, M., Raipuria, N., & Agrawal, M. K. (2016). Antimicrobial activity of Nostoc calcicola (Cyanobacteria) isolated from central India against human pathogens. Asian Journal of Pharmaceutics, 10(4), S554–S559. https://doi.org/10.22377/ajp.v10i04.892
Biondi, N., Piccardi, R., Margheri, M. C., Rodolfi, L., Smith, G. D., & Tredici, M. R. (2004). Evaluation of Nostoc strain ATCC 53789 as a potential source of natural pesticides. Applied and Environmental Microbiology, 70, 3313–3320. https://doi.org/10.1128/AEM.70.6.3313-3320.2004
Virág, E., Molnár, Z., & Ördög, V. (2011). Application of algal biomass for enhanced acclimatization of orchids. Acta Biologica Szegediensis, 55(1), 179–181.
Hashtroudi, M. S., Shariatmadari, Z., Riahi, H., & Ghassempour, A. (2013). Analysis of Anabaena vaginicola and Nostoc calcicola from Northern Iran, as rich sources of major carotenoids. Food Chemistry, 136, 1148–1153. https://doi.org/10.1016/j.foodchem.2012.09.055
Zhao, C., Fu, Q., Song, W., Zhang, D., Ahati, J., Pan, X., Al-Misned, F. A., & Mortuza, M. G. (2015). Calcifying cyanobacterium (Nostoc calcicola) reactor as a promising way to remove cadmium from water. Ecological Engineering, 81, 107–114. https://doi.org/10.1016/j.ecoleng.2015.04.012
Rippka, R., Deruelles, J., Waterbury, B., Herdman, M., & Stanier, R. Y. (1979). Generic assignments, strain histories and properties of pure cultures of Cyanobacteria. Journal of General Microbiology, 111, 1–61. https://doi.org/10.1099/00221287-111-1-1
Touloupakis, E., Silva Benavides, A. M., Cicchi, B., & Torzillo, G. (2016). Growth and hydrogen production of outdoor cultures of Synechocystis PCC 6803. Algal Research, 16, 78–85. https://doi.org/10.1016/j.algal.2016.06.010
Lichtenthaler, H. K. (1987). Chlorophylls and carotenoids: Pigments of photosynthetic biomembranes. Methods in Enzymology, 148, 350–382. https://doi.org/10.1016/0076-6879(87)48036-1
Lauceri, R., Chini Zittelli, G., & Torzillo, G. (2019). A simple method for rapid purification of phycobiliproteins from Arthrospira platensis and Porphyridium cruentum biomass. Algal Research, 44, 101685. https://doi.org/10.1016/j.algal.2019.101685
Eilers, P. H. C., & Peeters, J. C. H. (1988). A model for the relationship between light intensity and the rate of photosynthesis in phytoplankton. Ecological Modelling, 42, 199–215. https://doi.org/10.1016/0304-3800(88)90057-9
Touloupakis, E., Cicchi, B., & Torzillo, G. (2015). A bioenergetic assessment of photosynthetic growth of Synechocystis sp. PCC 6803 in continuous cultures. Biotechnology & Biofuels, 8, 133. https://doi.org/10.1186/s13068-015-0319-7
Szabó, M., Parker, K., Guruprasad, S., Kuzhiumparambil, U., Lilley, R. M., Tamburic, B., Schliep, M., Larkum, A. W., Schreiber, U., Raven, J. A., & Ralph, P. J. (2014). Photosynthetic acclimation of Nannochloropsis oculata investigated by multi-wavelength chlorophyll fluorescence analysis. Bioresource Technology, 167, 521–529. https://doi.org/10.1016/j.biortech.2014.06.046
Khajepour, F., Hosseini, S. A., Ghorbani Nasrabadi, R., & Markou, G. (2015). Effect of light intensity and photoperiod on growth and biochemical composition of a local isolate of Nostoc calcicola. Applied Biochemistry and Biotechnology, 176(8), 2279–2289. https://doi.org/10.1007/s12010-015-1717-9
Dron, A., Rabouille, S., Claquin, P., Le Roy, B., Talec, A., & Sciandra, A. (2012). Light-dark (12:12) cycle of carbon and nitrogen metabolism in Crocosphaera watsonii WH8501: Relation to the cell cycle. Environmental Microbiology, 14(4), 967–981. https://doi.org/10.1111/j.1462-2920.2011.02675.x
Kaftan, D., Meszaros, T., Whitmarsh, J., & Nedbal, L. (1999). Characterization of photosystem II activity and heterogeneity during the cell cycle of the green alga Scenedesmus quadricauda. Plant Physiology, 120, 433–441. https://doi.org/10.1104/pp.120.2.433
Canonico, M., Konert, G., & Kaňa, R. (2020). Plasticity of cyanobacterial thylakoid microdomains under variable light conditions. Frontiers in Plant Science, 11, 586543. https://doi.org/10.3389/fpls.2020.586543
Deschoenmaeker, F., Facchini, R., Carlos, J., Pino, C., Bayon-Vicente, G., Sachdeva, N., Flammang, P., & Wattiez, R. (2016). Nitrogen depletion in Arthrospira sp. PCC 8005, an ultrastructural point of view. Journal of Structural Biology, 196, 385–393. https://doi.org/10.1016/j.jsb.2016.08.007
Prosperi, C. H. (1994). A cyanophyte capable of fixing nitrogen under high levels of oxygen. Journal of Phycology, 30, 222–224. https://doi.org/10.1111/j.0022-3646.1994.00222.x
Bergman, B., Gallon, J. R., Rai, A. N., & Stal, L. J. (1997). N2 fixation by non-heterocystous cyanobacteria. FEMS Microbiology Reviews, 19, 139–185. https://doi.org/10.1111/j.1574-6976.1997.tb00296.x
Meeks, J. C., Campbell, E. L., Summers, M. L., & Wong, F. C. (2002). Cellular differentiation in the cyanobacterium Nostoc punctiforme. Archives in Microbiology, 178, 395–403. https://doi.org/10.1007/s00203-002-0476-5
Campbell, E. L., Summers, M. L., Christman, H., Martin, M. E., & Meeks, C. J. (2007). Global gene expression patterns of Nostoc punctiforme in steady-state dinitrogen grown heterocyst-containing cultures and at single time points during the differentiation of akinetes and hormogonia. Journal of Bacteriology, 189, 5247–5256. https://doi.org/10.1128/JB.00360-07
Meeks, J. C., Wycoff, K. L., Chapman, J. S., & Enderlin, C. S. (1983). Regulation of expression of nitrate and dinitrogen assimilation by Anabaena species. Applied and Environmental Microbiology, 45, 1351–1359. https://doi.org/10.1128/aem.45.4.1351-1359.1983
Otero, A., & Vincenzini, M. (2003). Extracellular polysaccharide synthesis by Nostoc strains as affected by N source and light intensity. Journal of Biotechnology, 102(2), 143–152. https://doi.org/10.1016/s0168-1656(03)00022-1
Jindal, N., Singh, D., & Khattar, J. (2011). Kinetics and physico-chemical characterization of exopolysaccharides produced by the cyanobacterium Oscillatoria formosa. World Journal of Microbiology and Biotechnology, 27(9), 2139–2146. https://doi.org/10.1007/s11274-011-0678-6
Singh, S., & Das, S. (2011). Screening, production, optimization and characterization of cyanobacterial polysaccharide. World Journal of Microbiology and Biotechnology, 27(9), 1971–1980. https://doi.org/10.1007/s11274-011-0657-y
Rossi, F., & De Philippis, R. (2015). Role of cyanobacterial exopolysaccharides in phototrophic biofilms and in complex microbial mats. Life, 5, 1218–1238. https://doi.org/10.3390/life5021218
Serpa, R., & Calderón, A. (2006). Effect of different nitrogen sources on the carotenoid and chlorophyll content of four peruvian strains of Dunaliella salina Teod. Ecologia Aplicada, 5(1,2), 93–99. Doi: https://doi.org/10.21704/rea.v5i1-2.895
Li, X., Liang, Y., Li, K., Jin, P., Tang, J., Klepacz-Smółka, A., Ledakowicz, S., & Daroch, M. (2021). Effects of low temperature, nitrogen starvation and their combination on the photosynthesis and metabolites of Thermosynechococcus E542: A comparison study. Plants (Basel), 10(10), 2101. https://doi.org/10.3390/plants10102101
Vonshak, A., & Novoplansky, N. (2008). Acclimation to low temperature of two Arthrospira platensis (Cyanobacteria) strains involves down-regulation of PSII and improved resistance to photoinhibition. Journal of Phycology, 44(4), 1071–1079. https://doi.org/10.1111/j.1529-8817.2008.00546.x
Tóth, T. N., Chukhutsina, V., Domonkos, I., Knoppová, J., Komenda, J., Kis, M., Lénárt, Z., Garab, G., Kovács, L., Gombos, Z., & van Amerongen, H. (2015). Carotenoids are essential for the assembly of cyanobacterial photosynthetic complexes. Biochimica et Biophysica Acta BBA (Bioenergetics), 1847, 1153–1165. https://doi.org/10.1016/j.bbabio.2015.05.020
Nishiyama, Y., Allakhverdiev, S. I., & Murata, N. (2006). A new paradigm for the action of reactive oxygen species in the photoinhibition of photosystem II. Biochimica et Biophysica Acta, 1757, 742–749. https://doi.org/10.1016/j.bbabio.2006.05.013
Norena-Caro, D. A., Malone, T. M., & Benton, M. G. (2021). Nitrogen sources and iron availability affect pigment biosynthesis and nutrient consumption in Anabaena sp. UTEX 2576. Microorganisms, 9(2), 431. https://doi.org/10.3390/microorganisms9020431
Rabouille, S., Van de Waal, D. B., Matthijs, H. C. P., & Huisman, J. (2014). Nitrogen fixation and respiratory electron transport in the cyanobacterium Cyanothece under different light/dark cycles. FEMS Microbiology Ecology, 87(3), 630–638. https://doi.org/10.1111/1574-6941.12251
Torzillo, G., & Vonshak, A. (2013). Environmental stress physiology with reference to mass cultures. In A. Richmond & Q. Hu (Eds.), Handbook of microalgal culture: Applied phycology and biotechnology. Oxford: Wiley Blackwell.
Dubinsky, Z., Matsukawa, R., & Karube, I. (1995). Photobiological aspects of algal mass culture. Journal of Marine Biotechnology, 2, 61–65.
Ramus, J. (1981). The capture and transduction of light energy. In C. S. Lobban & M. J. Wynne (Eds.), The biology of Seaweeds botanical monographs (Vol. 17, pp. 458–492). JohnWiley & Sons.
Fisher, T., Minnaard, J., & Dubinsky, Z. (1996). Photoacclimation in the marine alga Nannochloropsis sp. (Eustigmatophyte): A kinetic study. Journal of Plankton Research, 18, 1797–1818. https://doi.org/10.1093/plankt/18.10.1797
Touloupakis, E., Tartari, G., Chini Zittelli, G., & Torzillo, G. (2020). Growth and photosynthetic performance of Chlamydopodium fusiforme cells grown in BG11 and Bristol media. Journal of Applied Phycology, 32, 145–152. https://doi.org/10.1007/s10811-019-01900-y
Ogawa, T., Suzuki, K., & Sonoike, K. (2021). Respiration interacts with photosynthesis through the acceptor side of photosystem I, reflected in the dark-to-light induction kinetics of chlorophyll fluorescence in the cyanobacterium Synechocystis sp. PCC 6803. Frontiers in Plant Sciences, 12, 717968. https://doi.org/10.3389/fpls.2021.717968
Fujimori, T., Higuchi, M., Sato, H., Aiba, H., Muramatsu, M., Hihara, Y., & Sonoike, K. (2005). The mutant of sll1961, which encodes a putative transcriptional regulator, has a defect in regulation of photosystem stoichiometry in the cyanobacterium Synechocystis sp. PCC 6803. Plant Physiology, 139, 408–416. https://doi.org/10.1104/pp.105.064782
Ozaki, H., Ikeuchi, M., Ogawa, T., Fukuzawa, H., & Sonoike, K. (2007). Large-scale analysis of chlorophyll fluorescence kinetics in Synechocystis sp. PCC 6803: identification of the factors involved in the modulation of photosystem stoichiometry. Plant and Cell Physiology, 48(3), 451–458. https://doi.org/10.1093/pcp/pcm015
Falkowski, P. G., & Raven, J. A. (1997). Aquatic photosynthesis (p. 375). Blackwell Scientific.
Dubinsky, Z., Falkowski, P. G., & Wyman, K. (1986). Light harvesting and utilisation by phytoplankton. Plant and Cell Physiology, 27, 1335–1349. https://doi.org/10.1093/oxfordjournals.pcp.a077232
Chamizo, S., Adessi, A., Torzillo, G., & De Philippis, R. (2020). Exopolysaccharide features influence growth success in biocrust-forming cyanobacteria, moving from liquid culture to sand microcosms. Frontiers in Microbiology, 11, 568224. https://doi.org/10.3389/fmicb.2020.568224
Fasaei, F., Bitter, J. H., Slegers, P. M., & van Boxtel, A. J. B. (2018). Techno-economic evaluation of microalgae harvesting and dewatering systems. Algal Research, 31, 347–362. https://doi.org/10.1016/j.algal.2017.11.038
Norsker, N. H., Barbosa, M. J., Vermuë, M. H., & Wijffels, R. H. (2011). Microalgal production—a close look at the economics. Biotechnology Advances, 29(1), 24–27. https://doi.org/10.1016/j.biotechadv.2010.08.005
Acknowledgements
The work has been supported by the H2020 project “SABANA” funded by the European Union’s Horizon 2020 research and innovation program under grant agreement No 727874. The authors would like to thank Dr Gabriele Tartari for providing the phosphate and nitrate data, and Mr. Alvaro Andres Cerdas Ceden, CIMAR, University of Costa Rica, for pigment analysis.
Author information
Authors and Affiliations
Corresponding author
Ethics declarations
Conflict of interest
The authors declare that they have no conflict of interest.
Rights and permissions
Open Access This article is licensed under a Creative Commons Attribution 4.0 International License, which permits use, sharing, adaptation, distribution and reproduction in any medium or format, as long as you give appropriate credit to the original author(s) and the source, provide a link to the Creative Commons licence, and indicate if changes were made. The images or other third party material in this article are included in the article's Creative Commons licence, unless indicated otherwise in a credit line to the material. If material is not included in the article's Creative Commons licence and your intended use is not permitted by statutory regulation or exceeds the permitted use, you will need to obtain permission directly from the copyright holder. To view a copy of this licence, visit http://creativecommons.org/licenses/by/4.0/.
About this article
Cite this article
Touloupakis, E., Zittelli, G.C., Benavides, A.M.S. et al. Growth and photosynthetic performance of Nostoc linckia (formerly N. calcicola) cells grown in BG11 and BG110 media. Photochem Photobiol Sci 22, 795–807 (2023). https://doi.org/10.1007/s43630-022-00353-6
Received:
Accepted:
Published:
Issue Date:
DOI: https://doi.org/10.1007/s43630-022-00353-6