Abstract
Methamphetamine (METH), a commonly abused psychostimulant, causes dopamine neurotoxicity in humans, rodents, and nonhuman primates. This study examined the selective neuroanatomical pattern of dopaminergic neurotoxicity induced by METH in the mouse striatum. We examined the effect of METH on tyrosine hydroxylase (TH) and dopamine transporter (DAT) immunoreactivity in the different compartments of the striatum and in the nucleus accumbens. The levels of dopamine and its metabolites, 3,4-dihidroxyphenylacetic acid and homovanillic acid, as well as serotonin (5-HT) and its metabolite, 5-hydroxyindolacetic acid, were also quantified in the striatum. Mice were given three injections of METH (4 mg/kg, i.p.) at 3 h intervals and sacrificed 7 days later. This repeated METH injection induced a hyperthermic response and a decrease in striatal concentrations of dopamine and its metabolites without affecting 5-HT concentrations. In addition, the drug caused a reduction in TH- and DAT-immunoreactivity when compared to saline-treated animals. Interestingly, there was a significantly greater loss of TH- and DAT-immunoreactivity in striosomes than in the matrix. The predominant loss of dopaminergic terminals in the striosomes occurred along the rostrocaudal axis of the striatum. In contrast, METH did not decrease TH- or DAT-immunoreactivity in the nucleus accumbens. These results provide the first evidence that compartments of the mouse striatum, striosomes and matrix, and mesolimbic and nigrostriatal pathways have different vulnerability to METH. This pattern is similar to that observed with other neurotoxins such as MPTP, the most widely used model of Parkinson’s disease, in early Huntington’s disease and hypoxic/ischemic injury, suggesting that these conditions might share mechanisms of neurotoxicity.
Similar content being viewed by others
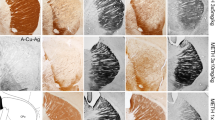
Avoid common mistakes on your manuscript.
Introduction
Methamphetamine (METH) is an illicit psychostimulant that is widely abused. The United Nations Office on Drugs and Crime (UNODC) conservatively estimates that there are between 15 and 16 million METH users worldwide, a figure similar to those for heroin and cocaine (UNODC 2008). The immediate effects of METH are associated with an increase in serotonin (5-HT) and dopamine (DA) concentrations in the synaptic cleft. METH exposure causes long-term neurotoxicity in rodents, nonhuman primates, and humans, and although some serotonergic loss is reported, mainly in rats (Wagner et al. 1980; Green et al. 1992; Nowak et al. 2007; Bortolato et al. 2009; Cadet et al. 2009), it predominantly affects the dopaminergic system (Seiden et al. 1976; Wagner et al. 1980; McCann et al. 1998; Melega et al. 2000; Cadet et al. 2007; Daberkow et al. 2008; Krasnova and Cadet 2009). In mice, repeated administration of METH produces neurodegeneration of dopamine axon terminals in the striatum and cell body loss in the substantia nigra (Sonsalla et al. 1996; Hirata and Cadet 1997). Damage has been demonstrated both histologically (O’Callaghan and Miller 1994) and biochemically (Sonsalla et al. 1989; Mann et al. 1997; Itzhak et al. 2000; Hogan et al. 2000; Staal et al. 2000; Nowak et al. 2007) and is reflected as a substantial decrease in the concentration of dopamine and its metabolites, a loss in the density of plasmalemmal and vesicular dopamine transporters, and a decrease in tyrosine hydroxylase (TH) activity. The mechanisms underlying this neurodegeneration are not fully understood currently, but a considerable body of data indicates that reactive oxygen and nitrogen species play an essential role (Itzhak et al. 1998, 2000; Deng and Cadet 1999; Imam et al. 2001; Krasnova and Cadet 2009).
We have recently found that the dopaminergic terminal loss induced in the striatum by MDMA, another amphetamine derivative compound, occurs predominantly in the striosomes compared to the matrix (Granado et al. 2008a). Interestingly, this pattern of dopaminergic terminal neurotoxicity is similar to that shown in early stages of MPTP-induced neurotoxicity in monkeys (Iravani et al. 2006) and in the adult weaver mouse (Graybiel et al. 1990). A predominant loss of TH in the striosomes has also been shown in a transgenic mouse model of dopa-responsive dystonia (Sato et al. 2008). Remarkably, this pattern not only applies to dopaminergic terminals but also extends to medium spiny neurons within the striosomal compartment, as found in early stages of Huntington disease (Hedreen and Folstein 1995) and after forebrain hypoxic-ischemic injury (Burke and Baimbridge 1993). Interestingly, these neurons are dopaminoceptive: they are innervated by dopaminergic terminals and express D1 or D2 dopamine receptors.
The striosome/matrix cytoarchitectonic organization of the striatum plays a central role in the functional organization of the striatum. Because of their connections with the limbic system, striosomes are functionally associated with reward-related behaviors (White and Hiroi 1998) and stimulant-induced neuronal events (Moratalla et al. 1996). The matrix is connected to sensorimotor regions of the brain and is more closely associated with normal motor functions (Berretta et al. 1997; Brown et al. 2002). Given the functional importance of the striosomes/matrix organization, an imbalance in the physiological dopaminergic activity or in differential signaling efficacy between these two compartments has been associated with the pathogenesis of abnormal and persistent motor behaviors and pathological reinforcement induced by repetitive administration of dopaminergic drugs (Moratalla et al. 1996; Hiroi et al. 2002; Sato et al. 2008; Crittenden et al. 2009; Darmopil et al. 2009).
To investigate whether the differential vulnerability of these two compartments to METH toxicity underlies the pathological consequences of METH abuse, we studied the relative susceptibility of the striatal compartments to the neurotoxic effects of METH by evaluating changes in the expression of two dopaminergic nigrostriatal markers, TH and dopamine transporter (DAT), 7 days after repeated METH administration. Our results show that striosomes are more vulnerable to METH than matrix.
Experimental Procedures
Animals and Treatment
Adult female C57BL/6J mice (20–25 g, Harlan Iberica, Barcelona, Spain) were housed in groups of 4–6 per cage at the Instituto Cajal in conditions of constant temperature at 21 ± 2°C, in a 12 h light/dark cycle, with free access to food and water. All experimental procedures were approved by the Bioethics Committee of the Instituto Cajal (following DC86/609/EU).
Mice received three injections of METH (4 mg/kg, i.p.) with 3 h intervals between injections. Control mice were given saline. Doses are expressed as free base. METH was obtained from Sigma-Aldrich (Madrid, Spain).
Measurement of Rectal Temperature
Rectal temperature was measured using a digital readout thermocouple (BAT-12 thermometer, Physitemp Instruments, Clifton, NJ, USA) with a resolution of 0.1°C and accuracy of ±0.1°C attached to a RET-3 Rodent Sensor. The sensor was inserted 2 cm into the mouse rectum while the mouse was lightly restrained by holding in the hand. A steady readout was obtained within 10 s of probe insertion. Temperature readings were taken every 30 min immediately before and after METH injections and hourly thereafter.
Immunohistochemistry
Seven days after the administration of METH (4 mg/kg, i.p. 3×), animals were deeply anesthetized (50 mg/kg, sodium pentobarbital) and transcardially perfused with 4% paraformaldehyde. Immunostaining was carried out in free-floating brain sections (30 μm) with standard avidin–biotin immunohistochemical protocols (Granado et al. 2008b, c; Martín et al. 2008; Rodrigues et al. 2007), with specific TH antisera (1:1,000; Chemicon International, Temecula, CA, USA), DAT monoclonal antibody (1:5,000; Chemicon International), and a μ opioid receptor (MOR-1) antisera (1:10,000; Chemicon International). After incubation with the primary (overnight) and secondary antisera (1 h; Vector Laboratories), peroxidase reactions were developed in diaminobenzidine.
Quantification of TH and DAT striatal expression was performed with the aid of an image analysis system (AIS, Imaging Research Inc., Linton, England) using a 5× lens. The proportional TH- or DAT-stained area was determined in each visible striosome and in its surrounding matrix, through the rostrocaudal axis of the striatum (Granado et al. 2008a, b). The data are presented as the proportional stained area (total TH- or DAT-positive area/scan area) in the striatal compartments in control and METH-treated animals. Measurements were carried out in five animals per treatment (5–6 sections/animal). The proportional TH- or DAT-immunostained area in the whole striatum was also measured (Granado et al. 2008b).
Measurement of Monoamines and Metabolites in Striatum
Seven days after treatment, the mice were killed by cervical dislocation, the brains were rapidly removed, and the striatum dissected out on ice. Dopamine, serotonin, and the metabolites—3,4-dihydroxyphenylacetic acid (DOPAC), homovanillic acid (HVA), and 5-hydroxyindolacetic acid (5-HIAA)—were measured by high-performance liquid chromatography and electrochemical detection. The mobile phase consisted of KH2PO4 (0.05 M), octanesulfonic acid (0.4 mM), EDTA (0.1 mM), and methanol (16%) and was adjusted to pH 3 with phosphoric acid, filtered, and degassed. The flow rate was 1 ml/min. The high-performance liquid chromatography system consisted of a pump (Waters 510) linked to an automatic sample injector (Loop 200 μl, Waters 717 plus Autosampler) and a stainless steel reversed-phase column (Spherisorb ODS2, 5 μm, 150 × 4.6 mm2; Waters, Milford, MA, USA) with a pre-column and a coulometric detector (Coulochem II; Esa, Chelmsford, MA, USA). The working electrode potential was set at 400 mV with a gain of 1 μA (for dopamine) and 500 nA (for the remaining compounds). The current produced was monitored by means of integration software (Unipoint; Gilson, Villier Le Bel, France).
Statistics
Data are presented as mean ± standard error of the mean (S.E.M.). The results of rectal temperature measurements were analyzed using one-way analysis of variance (ANOVA) for repeated measures.
Data obtained from image analysis of striatal TH and DAT immunostaining were analyzed using one-way ANOVA. Relevant differences were analyzed pairwise by post hoc comparisons with Tukey’s test or Dunn’s test to determine specific group differences. P values less than 0.05 were considered statistically significant.
All statistical analyses were performed using SigmaStat 2.03 program, and graphical representations were obtained using SigmaPlot 9.0 software.
Results
METH Induces Hyperthermia
METH treatment (4 mg/kg, i.p., three injections given at 3 h intervals) resulted in significant changes in rectal temperatures (Fig. 1). The first injection of METH induced a marked hypothermic response (1.2°C below that of saline-treated mice), peaking at 30 min and lasting at least 1 h. The second and third injections caused transient hyperthermia (1–1.5°C above saline) which reached a peak 30 min after each injection. The highest value was reached after the second injection of METH reaching 39.2°C. Statistical comparison revealed a significant difference between the response of mice to METH and to saline within the first 60 min following the first, second, and third injections (P < 0.001 as by one-way ANOVA RM) (Fig. 1).
Effect of METH on mouse rectal temperature. Repeated METH administration (4 mg/kg, i.p., every 3 h, 3×) produced a decrease in rectal temperature of the animals after the first injection and an increase in the rectal temperature, peaking 30 min after the second and third injections. The arrows indicate the time of drug injection. Data represent mean ± S.E.M., n = 6 animals/group
METH-Induced TH Loss in Mouse Striatum
Seven days after METH administration, immunohistochemistry revealed a marked overall decrease in density of TH-immunoreactive fibers in the striatum (Fig. 2) compared to the intense TH staining in saline-treated animals (Fig. 2a–f). Furthermore, in the caudoputamen of animals treated with METH, we saw patches or pockets with severe loss of TH-positive fibers compared with the rest of striatum. Although the TH fiber loss was similar along the rostrocaudal axis of the caudoputamen, we observed a significantly greater loss in the lateral part (Fig. 2d–f), suggesting that the motor areas are more vulnerable to METH than associative or limbic areas of the striatum. Quantitative image analysis revealed a significant decrease of 37.3% in TH-ir fibers within the caudoputamen of drug-treated animals 7 days after METH administration compared to saline-treated controls (P < 0.001) (Fig. 2g).
Effect of METH on TH-immunoreactivity in the striatum. Photomicrographs of striatal sections from mice treated with saline (a–c) or METH (d–f) stained for TH. Animals were killed 7 days after treatment. Note the TH-ir loss in the METH-treated mice along the rostrocaudal axis of the striatum. (g) Histograms of the proportional stained area of TH-ir. METH (4 mg/kg, i.p., every 3 h, 3×) produced a reduction in TH-immunoreactivity levels. Data represent mean ± S.E.M., n = 6 animals/group. * P < 0.001 vs. saline, one-way ANOVA. Bar 500 μm
DAT Loss in the Striatum of METH-Treated Mice
To confirm that TH fiber loss reflected terminal damage and not just a reduction in TH synthesis, we used immunohistochemistry to evaluate the expression of dopamine transporter (DAT). DAT is located on dopaminergic terminals and is expressed independently of TH synthesis or accumulation. METH also produced a significant loss of striatal DAT-positive fibers 7 days after injection compared with saline-treated mice (Fig. 3). As expected, the pattern of DAT-ir loss paralleled the pattern of TH-ir loss, with a similar decrease in DAT-ir fibers along the rostrocaudal axis, and a greater loss of DAT-ir fibers in striosomes and in the lateral striatum (Fig. 3a–f). Quantification of DAT-ir by image analysis revealed a significant decrease of 67% in METH-treated mice compared to saline-treated animals (P < 0.001) (Fig. 3g).
METH produces a decrease in DAT-immunoreactivity in the striatum. Photomicrographs of striatal sections from mice sacrificed 7 days after treatment with saline (a–c) or METH (d–f) stained for DAT. Note the DAT-ir loss in the METH-treated mice along the rostrocaudal axis of the striatum. (g) Histograms show the proportional stained area of DAT-immunoreactivity. METH (4 mg/kg, i.p., every 3 h, 3×) produced a reduction in DAT-immunoreactivity levels. Data represent mean ± S.E.M., n = 6 animals/group; * P < 0.001 vs. saline, one-way ANOVA. Bar 500 μm
Striosomes Are More Vulnerable to METH Neurotoxicity Than Matrix
Interestingly, in METH-treated animals, we also found that TH- and DAT-ir loss was greater in patches than in the rest of striatum (Fig. 4). To determine if these patches of highly damaged striatum corresponded to the location of striosomes, we performed immunostaining for MOR-1, a striosomal marker, in sections adjacent to those stained with TH or DAT. Areas immunostained with MOR-1 corresponded in number, size, and shape to the patches of greatest TH and DAT fiber loss (Fig. 4a–c). In the most damaged striatal areas, like the lateral part, this striosome/matrix pattern in TH/DAT staining was no longer evident due to the greater TH and DAT loss in these areas.
TH- and DAT-ir loss occurs predominantly in striosomes. Serially adjacent sections from a mouse treated with METH were stained for TH (a), MOR-1 (b), and DAT (c). Most patches of weak TH-ir matched patches of weak DAT-ir and both areas corresponded with striosomes identified by MOR-1 immunostaining. a′–c′ show an example of a striosome at higher magnification. Bar 500 μm (a–c) and 200 μm (a′–c′)
To study the relative vulnerability of striosomes compared to the matrix, we quantified TH-ir in both striatal compartments: striosomes and matrix (Fig. 5). When we determined the proportional area stained by TH, we found significant differences between the METH- and saline-treated animals for both the striosomes and matrix in medial, central, and lateral areas of the striatum. We also found that the difference was greater for the striosomes (average 88%, P < 0.001) than for the matrix (average 53%, P < 0.01) in all areas; however, the difference in TH content between striosomes and matrix was greatest in the medial area and lowest in the lateral area. It should be noted that in METH-treated mice, there was greater overall damage in the lateral striatum compared to the medial and central matrix of striatum (P < 0.05, respectively) (Fig. 5). Similar data were obtained when DAT-ir quantifications were performed (data not shown).
Loss of TH-immunoreactivity is greater in striosomes than in the matrix compartment. Histograms represent the proportional stained area of TH-immunoreactivity in striosomes (stm) and matrix (mtx) in the medial, center, and lateral areas of the striatum. Striatal slices from mice treated with METH (three injections of 4 mg/kg i.p. at 3 h intervals) were compared with those from saline-treated animals. * P < 0.001 vs. saline; # P < 0.01 vs. matrix; & P < 0.05 vs. lateral matrix
Striatal Dopamine and 5-HT Concentrations in METH-Treated Mice
Seven days after METH administration (4 mg/kg, three times at 3 h intervals), there was a reduction (76%) in the striatal dopamine content (ng/g tissue) of METH-treated mice compared to saline (P < 0.001). The metabolites of dopamine, DOPAC and HVA, were also affected by treatment with METH. DOPAC was reduced by 64% (P < 0.001) and HVA levels by 59% (P < 0.001) in the METH-treated mice (Fig. 6). There were no differences between METH- and saline-treated animals in striatal 5-HT concentration or 5-HIAA concentration (P = 0.67 and P = 0.63, respectively) (Fig. 6).
Effect of METH on the striatal content of DA, DOPAC, and HVA. Amine levels were measured by HPLC in homogenates from striata of mice treated with METH (4 mg/kg) or saline. Mice were sacrificed 7 days after drug administration. Data represent the mean ± SEM, n = 6 animals/group. * 0.001 compared with control animals
METH Administration Does Not Affect TH-ir in the Nucleus Accumbens
In contrast to our finding that administration of METH produces a marked loss of TH and DAT fibers in the striatum, we found no change in TH- or DAT-ir in the nucleus accumbens (NAc) (Fig. 7). Quantitative image analysis revealed no significant difference in TH-ir in the NAc between METH- and saline-treated mice 7 days after METH administration (P = 0.12) (Fig. 7c). These studies demonstrate that METH selectively reduces dopaminergic terminals in the striatum, but not in the NAc.
The NAc does not exhibit METH-induced neurotoxicity. Photomicrographs of TH-immunoreactivity in the NAc of mice treated with saline (a) or METH (b). Animals were killed 7 days after treatment. c Histograms of the proportional stained area of TH-immunoreactivity. METH (4 mg/kg, i.p., every 3 h, 3×) produced no reduction in TH-immunoreactivity. Data represent mean ± S.E.M., P = 0.12; n = 6 animals/group. Bar 500 μm
Discussion
Previous studies have shown that acute administration of repeated doses of METH to mice induces a long-term neurotoxic effect reflected by selective loss of nigrostriatal dopamine markers. The overall aim of this study was to investigate whether the striosome and matrix compartments of the mouse striatum show differential vulnerability to METH as evidenced by differences in TH- and DAT-immunostaining. This study provides the first evidence that METH produces a greater loss of TH/DAT positive terminals in the striosomes than in the matrix, suggesting that the striosomes are differentially affected by METH. Furthermore, under our experimental conditions, METH produces no toxicity in the mesolimbic dopaminergic pathway.
Levels of TH- and DAT-immunoreactivity are commonly used indexes of dopaminergic terminal damage (Iravani et al. 2005; Pavón et al. 2006, Granado et al. 2008a, b; Darmopil et al. 2008, 2009), and decreased TH levels have been reported after treatment with dopaminergic neurotoxins including METH, MDMA, 6-OHDA, and MPTP (Moratalla et al. 1992; Fornai et al. 2004; Broening et al. 2005; Iravani et al. 2005; Pavón et al. 2006; Thomas et al. 2009). In this study, we have demonstrated a marked loss of TH expression in the striosomes of mice treated with METH. In addition, this decrease in TH expression is accompanied by a reduction in DAT, an important marker of functional dopaminergic nerve terminals. The decrease in DAT expression indicates that the decreased TH-ir is not due to a decrease in TH synthesis but rather due to neuronal damage of dopaminergic terminals. This is further supported by the reduction in the concentration of dopamine and its principle metabolites in the striatum of METH-treated mice.
Although the mechanism underlying the neurodegeneration of dopaminergic nerve terminals is not known, a substantial body of evidence indicates that oxygen and nitrogen reactive species play an essential role. A single injection of METH induces a rapid increase in 2,3-DHBA in the striatal dialysate lasting for at least 2 h, a clear indication that hydroxyl radicals are being formed (Battaglia et al. 2002). Oxygen reactive species could come from auto-oxidation of extravesicular dopamine producing hydrogen peroxide (H2O2) which forms hydroxyl radicals and superoxide anion (O2 −) (Simola et al. 2007). These species could, in turn, react with NO to generate the potent oxidant peroxynitrite (ONOO−) and other reactive oxygen radicals (Beckman 1991; Radi et al. 1991). METH increases 3-nitrotyrosine levels in mouse striatum, an index for ONOO− production, and this effect is absent in nNOS knockout mice or mice overexpressing Cu/Zn superoxide dismutase (SOD) (Imam et al. 2001). In addition, nNOS inhibitors completely prevent the loss of dopamine induced by METH (Itzhak et al. 2000; Sanchez et al. 2003), and the neurotoxic effects of METH in the striatum are attenuated in transgenic mice overexpressing Cu/Zn-SOD (Cadet et al. 1994; Hirata et al. 1996). The latter results are particularly relevant since SOD is more abundant in the matrix than in the striosomes (Medina et al. 1996; Zhang et al. 1994) and this distribution pattern tightly corresponds with the relative preservation of TH- and DAT-ir in the matrix following METH.
Therefore, it seems reasonable to propose that the increased susceptibility of the striosomal compartment to the damaging effects of METH may be related to a lower antioxidant capacity in striosomes than in matrix. It is likely that at higher doses of METH, the differential vulnerability between striosomes and matrix that we report here might be lost since the higher METH dose would overwhelm the protective capacities of both compartments.
A similar pattern of greater striosomal damage in the striatum has been observed following the administration of other neurotoxins such as MDMA (Granado et al. 2008a, b) and MPTP (Iravani et al. 2005), or NMDA receptor agonists such as quinolinic acid (Figueredo-Cardenas et al. 1998). It is also seen in early Huntington’s disease (Hedreen and Folstein 1995) and following ischemia/reperfusion injury (Burke and Baimbridge 1993; Medina et al. 1996). Given the role of free radicals in cell damage (Fiskum et al. 1999), the difference in SOD levels between the striatal compartments may offer at least a partial explanation for the differential vulnerability observed in all these conditions. It suggests that common mechanisms may underlie the cell damage caused by these different neurotoxins and neurodegenerative diseases.
On the other hand, METH increases the extracellular concentration of glutamate in striatum (Nash and Yamamoto 1992; Stephans and Yamamoto 1994), and glutamate is involved in mediating the neurotoxic effects of METH by overactivating N-methyl-d-aspartate (NMDA) and alpha-amino-3-hydroxy-5-methyl-4-isoxazole propionic acid (AMPA) receptors, causing excitotoxicity. In fact, NMDA receptor antagonists block the long-term dopamine loss induced by METH (Fuller et al. 1992; Layer et al. 1993; Ohmori et al. 1993; Thomas and Kuhn 2005; Ke et al. 2008). AMPA and NMDA glutamate receptors have differential distribution in the striatal compartments. AMPA receptors containing GluR1 may predominate in striosomes, while AMPA receptors with GluR4 are more abundant in the matrix (Martin et al. 1993). NMDA receptors also appear to predominate in striosomes as suggested by in vitro studies showing that NMDA had a greater stimulatory effect on the release of dopamine in the striosomes than in matrix compartment (Gauchy et al. 1996). Thus, striosomes and matrix appear to express different glutamate receptors or receptor subunit combinations which could have consequences relevant to the neuropathology induced by METH. The idea that differences in glutamate receptor activation play a role in the degree of neurotoxicity in different striatal compartments is supported by reports that calbindin expression is associated with relative resistance to MPTP damage since high intracellular levels of calcium binding protein may protect the cell against excessive calcium entry (Burke and Baimbridge 1993). Interestingly, the calbindin distribution pattern in the striatum corresponds with the pattern of relative protection against METH-induced dopaminergic loss in the matrix.
In conclusion, this study indicates that in mice METH produces greater damage to the dopaminergic nerve terminals in the striosomes than to those in the matrix, where the mesolimbic dopaminergic pathway is relatively preserved. The neurotoxicity which leads to this greater vulnerability of the striosomes and the nigrostriatal dopaminergic pathway may involve factors that are common to other types of neuronal injury such as those produced by ischemia, excitotoxicity, MPTP, or HD. Further studies are necessary to evaluate the precise mechanisms involved in this drug-induced cell damage and to determine why striosomes are uniquely sensitive to METH and to other neurodegenerative diseases.
Abbreviations
- METH:
-
Methamphetamine
- PB:
-
Phosphate buffer
- PBST:
-
Phosphate sodium buffer with Triton X-100
- DA:
-
Dopamine
- TH:
-
Tyrosine hydroxylase
- DAT:
-
Dopamine transporter
- MOR-I:
-
Opioid μ receptor
- DOPAC:
-
3,4-Dihidroxyphenylacetic acid
- HVA:
-
Homovanillic acid
- 5-HT:
-
Serotonin
- 5-HIAA:
-
5-Hydroxyindolacetic acid
- MDMA:
-
3,4-Methylenedioxymethamphetamine
- MPTP:
-
1-methyl-4-phenyl-1,2,3,6-tetrahydropyridine
References
Battaglia G, Fornai F, Busceti CL, Aloisi G, Cerrito F, De Blasi A, Melchiorri D, Nicoletti F (2002) Selective blockade of mGlu5 metabotropic glutamate receptors is protective against methamphetamine neurotoxicity. J Neurosci 22:2135–2141
Beckman JS (1991) The double-edged role of nitric oxide in brain function and superoxide-mediated injury. J Dev Physiol 15:53–59
Berretta S, Parthasarathy HB, Graybiel AM (1997) Local release of GABAergic inhibition in the motor cortex induces immediate-early gene expression in indirect pathway neurons of the striatum. J Neurosci 17:4752–4763
Bortolato M, Frau R, Piras AP, Luesu W, Bini V, Diaz G, Gessa M, Ennas MG, Castelli MP (2009) Methamphetamine induces long-term alterations in reactivity to environmental stimuli: correlation with dopaminergic and serotonergic toxicity. Neurotox Res 15:232–245
Broening HW, Morford LL, Vorhees CV (2005) Interactions of dopamine D1 and D2 receptor antagonists with D-methamphetamine-induced hyperthermia and striatal dopamine and serotonin reductions. Synapse 56:84–93
Brown T, de Groote C, Brotchie J (2002) Recent advances in the treatment of L-DOPA-induced dyskinesia. IDrugs 5:454–468
Burke RE, Baimbridge KG (1993) Relative loss of the striatal striosome compartment, defined by calbindin-D28k immunostaining, following developmental hypoxic-ischemic injury. Neuroscience 56:305–315
Cadet JL, Sheng P, Ali S, Rothman R, Carlson E, Epstein C (1994) Attenuation of methamphetamine-induced neurotoxicity in copper/zinc superoxide dismutase transgenic mice. J Neurochem 62:380–383
Cadet JL, Krasnova IN, Jayanthi S, Lyles J (2007) Neurotoxicity of substituted amphetamines: molecular and cellular mechanisms. Neurotox Res 11:183–202
Cadet JL, Krasnova IN, Ladenheim B, Cai NH, McCoy MT, Atianjoh FE (2009) Methamphetamine preconditioning: differential protective effects on monoaminergic systems in the rat brain. Neurotox Res 15:252–259
Crittenden JR, Cantuti-Castelvetri I, Saka E, Keller-McGandy CE, Hernandez LF, Kett LR, Young AB, Standaert DG, Graybiel AM (2009) Dysregulation of CalDAG-GEFI and CalDAG-GEFII predicts the severity of motor side-effects induced by anti-parkinsonian therapy. Proc Natl Acad Sci USA 106:2892–2896
Daberkow DP, Riedy MD, Kesner RP, Keefe KA (2008) Effect of methamphetamine neurotoxicity on learning-induced Arc mRNA expression in identified striatal efferent neurons. Neurotox Res 14:307–315
Darmopil S, Muneton-Gomez VC, de Ceballos ML, Bernson M, Moratalla R (2008) Tyrosine hydroxylase cells appearing in the mouse striatum after dopamine denervation are likely to be projection neurones regulated by L-DOPA. Eur J Neurosci 27:580–592
Darmopil S, Martín AB, Diego IR, Ares S, Moratalla R (2009) Genetic inactivation of dopamine D1 but not D2 receptors inhibits L-DOPA-induced dyskinesia and histone activation. Biol Psychiatry, in press
Deng X, Cadet JL (1999) Methamphetamine administration causes overexpression of nNOS in the mouse striatum. Brain Res 851:254–257
Figueredo-Cardenas G, Harris CL, Anderson KD, Reiner A (1998) Relative resistance of striatal neurons containing calbindin or parvalbumin to quinolinic acid-mediated excitotoxicity compared to other striatal neuron types. Exp Neurol 149:356–372
Fiskum G, Murphy AN, Beal MF (1999) Mitochondria in neurodegeneration: acute ischemia and chronic neurodegenerative diseases. J Cereb Blood Flow Metab 19:351–369
Fornai F, Lenzi P, Frenzilli G, Gesi M, Ferrucci M, Lazzeri G, Biagioni F, Nigro M, Falleni A, Giusiani M, Pellegrini A, Blandini F, Ruggieri S, Paparelli A (2004) DNA damage and ubiquitinated neuronal inclusions in the substantia nigra and striatum of mice following MDMA (ecstasy). Psychopharmacology (Berl) 173:353–363
Fuller RW, Hemrick-Luecke SK, Ornstein PL (1992) Protection against amphetamine-induced neurotoxicity toward striatal dopamine neurons in rodents by LY274614, an excitatory amino acid antagonist. Neuropharmacology 31:1027–1032
Gauchy C, Desban M, Glowinski J, Kemel ML (1996) Distinct regulations by septide and the neurokinin-1 tachykinin receptor agonist [pro9]substance P of the N-methyl-D-aspartate-evoked release of dopamine in striosome- and matrix-enriched areas of the rat striatum. Neuroscience 73:929–939
Granado N, Escobedo I, O’shea E, Colado MI, Moratalla R (2008a) Early loss of dopaminergic terminals in striosomes after MDMA administration to mice. Synapse 62:80–84
Granado N, O’Shea E, Bove J, Vila M, Colado MI, Moratalla R (2008b) Persistent MDMA-induced dopaminergic neurotoxicity in the striatum and substantia nigra of mice. J Neurochem 107:1102–1112
Granado N, Ortiz O, Suárez LM, Martín ED, Ceña V, Solís JM, Moratalla R (2008c) D1 but not D5 dopamine receptors are critical for LTP, spatial learning and LTP-induced arc and zif268 expression in the hippocampus. Cereb Cortex 18:1–12
Graybiel AM, Ohta K, Roffler-Tarlov S (1990) Patterns of cell and fiber vulnerability in the mesostriatal system of the mutant mouse weaver. I. Gradients and compartments. J Neurosci 10:720–733
Green AR, De Souza RJ, Williams JL, Murray TK, Cross AJ (1992) The neurotoxic effects of methamphetamine on 5-hydroxytryptamine and dopamine in brain: evidence for the protective effect of chlormethiazole. Neuropharmacology 31:315–321
Hedreen JC, Folstein SE (1995) Early loss of neostriatal striosome neurons in Huntington’s disease. J Neuropathol Exp Neurol 54:105–120
Hirata H, Cadet JL (1997) p53-knockout mice are protected against the long-term effects of methamphetamine on dopaminergic terminals and cell bodies. J Neurochem 69:780–790
Hirata H, Ladenheim B, Carlson E, Epstein C, Cadet JL (1996) Autoradiographic evidence for methamphetamine-induced striatal dopaminergic loss in mouse brain: attenuation in CuZn-superoxide dismutase transgenic mice. Brain Res 714:95–103
Hiroi N, Martín AB, Grande C, Alberti I, Rivera A, Moratalla R (2002) Molecular dissection of dopamine receptor signaling. J Chem Neuroanat 23:237–242
Hogan KE, Staal RGW, Sonsalla PK (2000) Analysis of VMAT2 binding after methamphetamine or MPTP treatment: disparity between homogenates and vesicle preparations. J Neurochem 74:2217–2220
Imam SZ, Newport GD, Itzhak Y, Cadet JL, Islam F, Slikker W, Ali SF (2001) Peroxynitrite plays a role in methamphetamine-induced dopaminergic neurotoxicity: evidence from mice lacking neuronal nitric oxide synthase gene or overexpressing copper-zinc superoxide dismutase. J Neurochem 76:745–749
Iravani MM, Syed E, Jackson MJ, Johnston LC, Smith LA, Jenner P (2005) A modified MPTP treatment regime produces reproducible partial nigrostriatal lesions in common marmosets. Eur J Neurosci 21:841–854
Iravani MM, Tayarani-Binazir K, Chu WB, Jackson MJ, Jenner P (2006) In 1-methyl-4-phenyl-1,2,3,6-tetrahydropyridine-treated primates, the selective 5-hydroxytryptamine 1a agonist (R)-(+)-8-OHDPAT inhibits levodopa-induced dyskinesia but only with increased motor disability. J Pharmacol Exp Ther 319:1225–1234
Itzhak Y, Gandia C, Huang PL, Ali SF (1998) Resistance of neuronal nitric oxide synthase-deficient mice to methamphetamine-induced dopaminergic neurotoxicity. J Pharmacol Exp Ther 284:1040–1047
Itzhak Y, Martin JL, Ali SF (2000) nNOS inhibitors attenuate methamphetamine-induced dopaminergic neurotoxicity but not hyperthermia in mice. Neuroreport 11:2943–2946
Ke JJ, Chen HI, Jen CJ, Kuo YM, Cherng CG, Tsai YP, Ho MC, Tsai CW, Yu L (2008) Mutual enhancement of central neurotoxicity induced by ketamine followed by methamphetamine. Toxicol Appl Pharmacol 227:239–247
Krasnova IN, Cadet JL (2009) Methamphetamine toxicity and messengers of death. Brain Res Rev 60:379–407
Layer RT, Bland LR, Skolnick P (1993) MK-801, but not drugs acting at strychnine-insensitive glycine receptors, attenuate methamphetamine nigrostriatal toxicity. Brain Res 625:38–44
Mann H, Ladenheim B, Hirata H, Moran TH, Cadet JL (1997) Differential toxic effects of methamphetamine (METH) and methylenedioxymethamphetamine (MDMA) in multidrug-resistant (mdr1a) knockout mice. Brain Res 769:340–346
Martin LJ, Blackstone CD, Huganir RL, Price DL (1993) The striatal mosaic in primates: striosomes and matrix are differentially enriched in ionotropic glutamate receptor subunits. J Neurosci 13:782–792
Martín AB, Fernández-Espejo E, Ferrer B, Gorriti MA, Navarro M, Rodríguez de Fonseca F, Moratalla R (2008) Expression and function of CB1 receptor in the rat striatum: localization and effects on D1 and D2 dopamine receptor-mediated motor behaviors. Neuropsychopharmacology 33:1667–1679
McCann UD, Wong DF, Yokoi F, Villemagne V, Dannals RF, Ricaurte GA (1998) Reduced striatal dopamine transporter density in abstinent methamphetamine and methcathinone users: evidence from positron emission tomography studies with [11C]WIN-35,428. J Neurosci 18:8417–8422
Medina L, Figueredo-Cardenas G, Rothstein JD, Reiner A (1996) Differential abundance of glutamate transporter subtypes in amyotrophic lateral sclerosis (ALS)-vulnerable versus ALS-resistant brain stem motor cell groups. Exp Neurol 142:287–295
Melega WP, Lacan G, Desalles AA, Phelps ME (2000) Long-term methamphetamine-induced decreases of [(11)C]WIN 35,428 binding in striatum are reduced by GDNF:PET studies in the vervet monkey. Synapse 35:243–249
Moratalla R, Quinn B, DeLanney LE, Irwin I, Langston JW, Graybiel AM (1992) Differential vulnerability of primate caudate-putamen and striosome-matrix dopamine systems to the neurotoxic effects of 1-methyl-4-phenyl-1,2,3,6-tetrahydropyridine. Proc Natl Acad Sci USA 89:3859–3863
Moratalla R, Xu M, Tonegawa S, Graybiel AM (1996) Cellular responses to psychomotor stimulant and neuroleptic drugs are abnormal in mice lacking the D1 dopamine receptor. Proc Natl Acad Sci USA 93:14928–14933
Nash JF, Yamamoto BK (1992) Methamphetamine neurotoxicity and striatal glutamate release: comparison to 3,4-methylenedioxymethamphetamine. Brain Res 581:237–243
Nowak P, Bortel A, Dabrowska J, Oswiecimska J, Drosik M, Kwiecinski A, Opara J, Kostrzewa RM, Brus R (2007) Amphetamine and mCPP effects on dopamine and serotonin striatal in vivo microdialysates in an animal model of hyperactivity. Neurotox Res 11:131–144
O’Callaghan JP, Miller DB (1994) Neurotoxicity profiles of substituted amphetamines in the C57BL/6J mouse. J Pharmacol Exp Ther 270:741–751
Ohmori T, Koyama T, Muraki A, Yamashita I (1993) Competitive and noncompetitive N-methyl-D-aspartate antagonists protect dopaminergic and serotonergic neurotoxicity produced by methamphetamine in various brain regions. J Neural Transm Gen Sect 92:97–106
Pavón N, Martín AB, Mendialdua A, Moratalla R (2006) ERK phosphorylation and FosB expression are associated with L-DOPA-induced dyskinesia in hemiparkinsonian mice. Biol Psychiatry 59:64–74
Radi R, Beckman JS, Bush KM, Freeman BA (1991) Peroxynitrite-induced membrane lipid peroxidation: the cytotoxic potential of superoxide and nitric oxide. Arch Biochem Biophys 288:481–487
Rodrigues TB, Granado N, Ortiz O, Cerdán S, Moratalla R (2007) Metabolic interactions between glutamatergic and dopaminergic neurotransmitter systems are mediated through D(1) dopamine receptors. J Neurosci Res 85:3284–3293
Sanchez V, Zeini M, Camarero J, O’Shea E, Bosca L, Green AR, Colado MI (2003) The nNOS inhibitor, AR-R17477AR, prevents the loss of NF68 immunoreactivity induced by methamphetamine in the mouse striatum. J Neurochem 85:515–524
Sato K, Sumi-Ichinose C, Kaji R, Ikemoto K, Nomura T, Nagatsu I, Ichinose H, Ito M, Sako W, Nagahiro S, Graybiel AM, Goto S (2008) Differential involvement of striosome and matrix dopamine systems in a transgenic model of dopa-responsive dystonia. Proc Natl Acad Sci USA 105:12551–12556
Seiden LS, Fischman MW, Schuster CR (1976) Long-term methamphetamine induced changes in brain catecholamines in tolerant rhesus monkeys. Drug Alcohol Depend 1:215–219
Simola N, Morelli M, Carta AR (2007) The 6-hydroxydopamine model of Parkinson’s disease. Neurotox Res 11:151–167
Sonsalla PK, Nicklas WJ, Heikkila RE (1989) Role for excitatory amino acids in methamphetamine-induced nigrostriatal dopaminergic toxicity. Science 243:398–400
Sonsalla PK, Jochnowitz ND, Zeevalk GD, Oostveen JA, Hall ED (1996) Treatment of mice with methamphetamine produces cell loss in the substantia nigra. Brain Res 738:172–175
Staal RGW, Hogan KA, Liang CL, German DC, Sonsalla PK (2000) In vitro studies of striatal vesicles containing the vesicular monoamine transporter (VMAT2): rat versus mouse differences in sequestration of 1-methyl-4-phenylpyridinium. J Pharmacol Exp Ther 293:329–335
Stephans SE, Yamamoto BK (1994) Methamphetamine-induced neurotoxicity: roles for glutamate and dopamine efflux. Synapse 17:203–209
Thomas DM, Kuhn DM (2005) MK-801 and dextromethorphan block microglial activation and protect against methamphetamine-induced neurotoxicity. Brain Res 1050:190–198
Thomas DM, Francescutti-Verbeem DM, Kuhn DM (2009) Increases in cytoplasmic dopamine compromise the normal resistance of the nucleus accumbens to methamphetamine neurotoxicity. J Neurochem 109:1745–1755
UNODC United Nations Office on Drugs and Crime (2008) Synthetic drugs on the rise. Perspectives 6:1–20
Wagner GC, Ricaurte GA, Seiden LS, Schuster CR, Miller RJ, Westley J (1980) Long-lasting depletions of striatal dopamine and loss of dopamine uptake sites following repeated administration of methamphetamine. Brain Res 181:151–160
White NM, Hiroi N (1998) Preferential localization of self-stimulation sites in striosomes/patches in the rat striatum. Proc Natl Acad Sci USA 95:6486–6491
Zhang P, Anglade P, Hirsch EC, Javoy-Agid F, Agid Y (1994) Distribution of manganese-dependent superoxide dismutase in the human brain. Neuroscience 61:317–330
Acknowledgments
This research was supported by grants from the Spanish Ministerios de Ciencia e Innovación y de Sanidad y Política Social, ISCIII: FIS PI07-1073, PNSD, RedRTA (RD06/0001/1011) and CIBERNED to RM and SAF2007-65175, FIS PI070892, PNSD 2006/1018, 2008/074; RTA06/0001/0006 to MIC and CIBERNED CB06/05/0065 to CVA. The authors would like to thank Emilia Rubio, Marco de Mesa and José María Caramés for their excellent technical assistance.
Open Access
This article is distributed under the terms of the Creative Commons Attribution Noncommercial License which permits any noncommercial use, distribution, and reproduction in any medium, provided the original author(s) and source are credited.
Author information
Authors and Affiliations
Corresponding author
Additional information
In memoriam to Dr. J.A. Burzaco for his dedication to Parkinson’s patients.
Rights and permissions
Open Access This is an open access article distributed under the terms of the Creative Commons Attribution Noncommercial License (https://creativecommons.org/licenses/by-nc/2.0), which permits any noncommercial use, distribution, and reproduction in any medium, provided the original author(s) and source are credited.
About this article
Cite this article
Granado, N., Ares-Santos, S., O’Shea, E. et al. Selective Vulnerability in Striosomes and in the Nigrostriatal Dopaminergic Pathway After Methamphetamine Administration. Neurotox Res 18, 48–58 (2010). https://doi.org/10.1007/s12640-009-9106-1
Received:
Revised:
Accepted:
Published:
Issue Date:
DOI: https://doi.org/10.1007/s12640-009-9106-1