Abstract
Martensitic steels (Aermet®100, Ferrium®M54™, Ferrium®S53®, and experimental CrNiMoWV at ultra-high yield strength of 1550 to 1725 MPa) similarly resist hydrogen environment assisted cracking (HEAC) in aqueous NaCl. Cracking is transgranular, ascribed to increased steel purity and rare earth addition compared to intergranular HEAC in highly susceptible 300M. Nano-scale precipitates ((Mo,Cr)2C and (W,V)C) reduce H diffusivity and the K-independent Stage II growth rate by 2 to 3 orders of magnitude compared to 300M. However, threshold K TH is similarly low (8 to 15 MPa√m) for each steel at highly cathodic and open circuit potentials. Transgranular HEAC likely occurs along martensite packet and {110}α′-block interfaces, speculatively governed by localized plasticity and H decohesion. Martensitic transformation produces coincident site lattice interfaces; however, a connected random boundary network persists in 3D to negate interface engineering. The modern steels are near-immune to HEAC when mildly cathodically polarized, attributed to minimal crack tip H production and uptake. Neither reduced Co and Ni in M54 and CrNiMoWV nor increased Cr in S53 broadly degrade HEAC resistance compared to baseline AM100. The latter suggests that crack passivity dominates acidification to widen the polarization window for HEAC resistance. Decohesion models predict the applied potential dependencies of K TH and da/dt II with a single-adjustable parameter, affirming the importance of steel purity and trap sensitive H diffusivity.

















Similar content being viewed by others
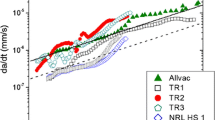
Notes
Two ultra-high strength martensitic stainless steels complete this alloy sequence: Custom®465 (0.01C-11.4Cr-10.9Ni-0.9Mo-0.7W-1.6Ti; wt pct) and Ferrium®PH48S™ (0.02C-10.9Cr-8.6Ni-7.3Co-1.6Mo-0.1W-0.6Ti-0.4Al; wt pct) strengthened by precipitation of Ni3Ti or Ni3(Ti,Al) plus NiAl, respectively. The HEAC behavior and microstructures of these steels are reported elsewhere, with emphasis on high yield strengths of 1600 to 1700 MPa.[48,49]
All potentials are reported with respect to the saturated calomel electrode (SCE).
Fully percolated interface cracking at the lower-bound f special is replaced with an increasing fraction of ductile cracking as f special rises to the upper limit. Given the f special for each UHSS in Table IV, this result suggests that fractographic interpretation of cracking through martensite must consider the presence of micro-meter scale regions of plastic-tearing fracture dispersed among the block and packet interfaces that are susceptible to H damage.
Wang et al. argue that H partitions to thin-film austenite, which lowers H concentration at martensite interfaces to enhance H cracking resistance.[87] This mechanism is not relevant for HEAC, which is governed by open system H concentration where all trap states are independently filled without partition.[88]
XRD yielded 1.97 vol pct austenite in commercially processed AM100 (Tables II and III specimens) and 1.52 vol pct for aged-LN2 chilled steel (5 specimens). The aged-chilled specimens that exhibited HEAC contained 1.47 vol pct austenite, while the HEAC-resistant aged-chilled specimens contained 1.60 vol pct austenite.
Previous publications contained a typographical error where (1 − C L) was presented rather than the correct form of (1 − C Lσ ) in the denominator of the second-bracketed term.[11,14,43,90] (1 − C Lσ ) was used in all calculations, and moreover (1 − C Lσ ) cancels in Eq. [3], given the values of E B, C L, and C RT for UHSS in NaCl with −1100 mV < E app < −400 mV.
C Hσ-crit is calculated in the context of crack tip σ H enhancement and is large for the stress field from SGP analysis[14] or the dislocation model (Eq. [1]).[96] With σ H = 12 GPa (7σ YS ) for AM100 and M54, C H-Diff of 1 wppm is elevated (Eq. [4]) to C Hσ of 17,200 wppm (0.49 atom fraction H). This high H content could lead to second-order σ H relaxation and perhaps hydride formation at the embrittled interface. Only limited experimental evidence supports very high crack tip H concentration for an Al alloy; the micro-meter scale FPZ and mobility of H challenge this measurement.[10]
The vendor reported yield strength of 300M (1580 MPa, Table I) is low compared to a published σ YS of 1787 MPa for this same austenitization and temper.[23] Modeling using this higher strength yields identical results compared to Figure 17, except k IG is 1.00 MPa√m for a reasonable interface toughness of 89 MPa√m, α is 2.0 MPa√m (atom frac H)−1, and the calculated C Hσ-crit is 1140 wppm. The interpretation that follows is unchanged.
The 120 and 250-fold decreases in predicted da/dt II for AM100 compared to 300M, shown in Figure 17(b) for the lowest and highest E app, respectively, are governed by: (a) an 80-fold decrease in D H-eff for AM100 and (b) the squared inversed error function term, decreased from 1.3 for 300M to 0.3 for AM100 at E app of −550 mV, and from 1.8 to 1.0 at −1100 mV.
References
R.P. Gangloff: in Compr. Struct. Integr., vol. 6, I. Milne, R.O. Ritichie, B. Karihaloo, J. Petit, and P. Scott, eds., Elsevier Science, New York, 2003, pp. 31–101.
W.M. Garrison, Jr. and N.R. Moody: in Gaseous Hydrog. Embrittlement Mater. Energy Technol., vol. 1, R.P. Gangloff and B.P. Somerday, eds., Woodhead Publishing Limited, Cambridge, 2012, pp. 421–92.
D.P. Dautovich and S. Floreen: in Hydrogen Embrittlement of Iron Based Alloys, R.W. Staehle, J. Hochmann, R.D. McCright, and J.E. Slater, eds., NACE International, Houston, 1973, pp. 798–815.
C.J. McMahon: Engr. Fract. Mech., vol. 68, 2001, pp. 773-88.
R.P. Wei: Fracture Mechanics: Integration of Mechanics, Materials Science and Chemistry, Cambridge University Press, New York, NY, 2010.
R.A. Oriani: Corrosion Journal, vol. 43, 1987, pp. 390-7.
I.M. Robertson, P. Sofronis, A. Nagao, M.L. Martin, S. Wang, D.W. Gross and K.E. Nygren: Metall. Mater. Trans. A, vol. 46A, 2014, pp. 2323-41.
S.P. Lynch: Corros. Rev., vol. 30, 2012, pp. 105-123.
R.P. Gangloff: in Hydrog. Eff. Mater. Behav. Corros. Deform. Interact., N.R. Moody, A.W. Thompson, R.E. Ricker, G.W. Was, and R.H. Jones, eds., The Minerals Metals & Materials Society, Warrendale, PA, 2003, pp. 477–97.
R.P. Gangloff: in Materials Performance in Hydrogen Environments, B.P. Somerday and P. Sofronis, eds., ASME, New York, 2017.
R.P. Gangloff, H. M. Ha, J.T. Burns, and J.R. Scully: Metall. Mater. Trans. A, vol 45, 2014, pp. 3814-34.
W.W. Gerberich: in Gaseous Hydrog. Embrittlement Mater. Energy Technol., vol. 2, R.P. Gangloff and B.P. Somerday, eds., Woodhead Publishing Limited, Cambridge, 2012, pp. 209–46.
B.A. Kehler and J.R. Scully: Corrosion Journal, vol. 65, 2008, pp. 465-77.
E. Martinez-Paneda, C.F. Niordson and R.P. Gangloff: Acta Mater., vol 117, 2016, pp. 321-32.
N. Bandyopadhyay, J. Kameda, and C.J. McMahon: Metall. Trans. A, vol. 14, 1983, pp. 881-8.
C.J. McMahon: Gaseous Hydrog. Embrittlement Mater. Energy Technol., vol. 2, R.P. Gangloff and B.P. Somerday, eds., Woodhead Publishing Limited, Cambridge, 2012, pp. 154–65.
G.B. Olson: Acta Mater., vol. 61, 2013, pp. 771-81.
C.J. Kuehmann and G.B. Olson: Mater. Sci. Technol., vol. 25, 2009, pp. 472-8.
C.E. Campbell and G.B. Olson: J. Comput. Mater. Des., vol. 7, 2001, pp. 145-70.
C.E. Campbell and G.B. Olson: J. Comput. Mater. Des., vol. 7, 2001, pp. 171-94.
G.R. Speich and W.C. Leslie: Metall. Trans., vol. 3, 1972, pp. 1043-54.
G.R. Speich, D. Dabkowski, and L. Porter: Metall. Trans. A, vol. 4, 1973, pp. 303-15.
R.O. Ritchie, M.O. Castro Cedeno, V.F. Zackay, and E.F. Parker: Metall. Trans. A, vol. 9, 1978, pp. 35-40.
Y.H. Kim, H.J. Kim, and J.W. Morris: Metall. Trans. A, vol. 17A, 1986, pp. 1157-64.
Y. Lee and R.P. Gangloff: Metall. Mater. Trans. A, vol. 38, 2007, pp. 2174-90.
D. Figueroa and M.J. Robinson: Corros. Sci., vol. 52, 2010, pp. 1593-1602.
R. Ayer and P.M. Machmeier: Metall. Trans. A, vol. 24, 1993, pp. 1943-55.
R. Padmanabhan: Ph.D. Dissertation, Oregon Graduate Center, Beaverton, OR, 1982.
R. Padmanabhan and W.E. Wood: Corrosion, vol. 41, 1985, pp. 688-99.
R.L.S. Thomas, J.R. Scully, and R.P. Gangloff: Metall. Trans. A, vol. 34, 2003, pp 327-44.
R.L.S. Thomas, D. Li, R.P. Gangloff, and J.R. Scully: Metall. Mater. Trans. A, vol. 33, 2002, pp. 1991-2004.
Processing Information for Ferrium M54, QPI-M54, QuesTek Innovations, Evanston, IL, 2012.
H.-J. Jou: US Patent, No. US9051635 B2, 2015.
J. Waldman, W.M. Garrison, Jr., and R.P. Gangloff: Final Report 0032-11/CD002, STTR Topic No. N08-T010, Navmar Applied Sciences Corporation, Warminster, PA, 2011.
W.M. Garrison, Jr.: US Patent Application, No. US2013/0228253, 2013.
Processing Information for Ferrium S53, QPI-S53, QuesTek Innovations, Evanston, IL, 2013.
C.J. Kuehmann, G.B. Olson, and J-J Jou: US Patent, No. US7967927 B2, 2011.
C.J. Kuehmann, B.E. Tufts, and P. Trester: Adv. Mater. Process., vol. 166, 2008, pp. 37-40.
R.P. Gangloff and A.Turnbull: in Model. Environ. Eff. Crack Initiat. Propag., W.W. Gerberich and R.H. Jones, eds., TMS-AIME, Warrendale, 1986, pp. 55–81.
F.G. Wei and K. Tsuzaki: in Gaseous Hydrog. Embrittlement Mater. Energy Technol. vol. 1, R.P. Gangloff and B.P. Somerday, eds., Woodhead Publishing Limited, Cambridge, 2012, pp. 493–525.
J. Wright: in Proc. 2010 Stainless Steel Work Am. Conf., Stainless Steel World, Woodlands, Houston, TX, 2010, pp. 1–13.
J. Grabowski, C. Kuehmann, J. Sebastian, H.-J. Jou, R. Kooy, and C. Kern: in Proc. 2012 Aircraft Airworth. Sustain. Conf., Baltimore, MD, 2012, pp. 1–23.
R.P. Gangloff, Corrosion Journal, vol. 72, 2016, pp, 862-80.
The Minerals, Metals and Materials Society: Modeling Across Scales: A Roadmapping Study for Connecting Materials Models and Simulations Across Length and Time Scales, The Minerals, Metals and Materials Society, Warrendale, PA, 2015, doi:10.7449/multiscale_1.
Y.H. Kim and J.W. Morris Jr.: Metall. Mater. Trans. A, vol. 14, 1983, pp. 1883-8.
S. Bechtle, M. Kumar, B.P. Somerday, M.E. Launey, and R.O. Ritchie: Acta Mater., vol. 57, 2009, pp. 4148-57.
S. Kobayashi, T. Maruyama, S. Tsurekawa, and T. Watanabe: Acta Mater., vol. 60, 2012, pp. 6200-12.
G.L. Pioszak: Ph.D. Dissertation, University of Virginia, Charlottesville, VA, 2015.
G.L. Pioszak and R.P. Gangloff: Corros. J., 2017. DOI:10.5006/2437.
R. Chait: Metall. Trans., vol. 3, 1972, pp. 369-75.
C.H. Yoo, H.M. Lee, J.W. Chan, and J.W. Morris: Metall. Mater. Trans. A, vol. 27, 1996, pp. 3466-72.
C. Wang, C. Zhang, and Z Yang: Micron, vol. 67, 2014, pp. 112-16.
F.J. Humphreys: Scripta Mater., vol. 51, 2004, pp. 771-6.
F.J. Humphreys: J. Mater. Sci., vol. 6, 2001, pp. 3833-54.
D. G. Brandon: Acta Metall., vol. 14, 1966, pp. 1479-84.
P. Lejcek: Grain Bound Segregation in Metals, Springer, Prague, 2010, pp. 5–22.
E.E. Underwood: Quantitative Stereology, Addison-Wesley, Reading, MA, 1970.
E. Charkaluk, M. Bigerelle, and A. Iost: Eng. Fract. Mech., vol. 61, 1998, pp. 119-39.
C.B. Crane and R.P. Gangloff: Corros. J., vol. 72, 2016, pp. 221-41.
R.P. Gangloff, D.C. Slavik, R.S. Piascik, and R.H. Van Stone: in Small Crack Test Methods, ASTM STP 1149, J. M. Larsen and J. E. Allison, eds., ASTM International, West Conshohocken, PA, 1992, pp 116–68.
J.K. Donald and J. Ruschau: in Fatigue Crack Meas. Tech. Appl., K.J. Marsh, R.A. Smith, and R.O. Ritchie, eds., EMAS, West Midlands, 1991, pp. 11–38.
C.C. Kinney, K.R. Pytlewski, A.G. Khachaturyan, and J.W. Morris, Jr.: Acta Mater., vol. 69, 2014, pp. 372-85.
S. Morito, H. Tanaka, R. Konishi, T. Furuhara, and T. Maki: Acta Mater., vol. 51, 2003, pp. 1789-99.
S. Morito, X. Huang, T. Furuhara, T. Maki, and N. Hansen: Acta Mater., vol 54, 2006, pp. 5323-31.
J.W. Morris: ISIJ International, vol. 51, 2011, pp. 1569-75.
R. Bhambroo, S. Roychowdhury, V. Kain, and V.S. Raju: Matls. Sci. Engr. A, vol. 568, 2013 pp.127-33.
M. Gao and R.P. Wei: Acta Metall., vol. 32, 1984, pp. 2115-24.
A. Shibata, H. Takahashi, and N. Tsuji: ISIJ Intl., vol. 52, 2012, pp. 208-12.
A. Nagao, C.D. Smith, M. Dadfarnia, P. Sofronis, and I.M. Robertson: Acta Mater., vol. 60, 2012, pp. 5182-9.
A. Nagao, C.D. Smith, M. Dadfarnia, P. Sofronis, and I.M. Robertson: Procedia Mater. Sci., vol. 3, 2014, pp. 1700-5.
E.U. Lee, M. Stanley, B. Pregger, C. Lei and E. Lipnickas: Naval Air Warfare Center Aircraft Division, Report No. NAWCADPAX/TIM-2014/292, Patuxent River, MD, 2015.
C.J. McMahon: Interface Sci., vol. 12, 2004, pp. 141-6.
N. Bandyopadhyay and C.J. McMahon: Metall. Trans. A, vol 14, 1983, pp. 1313-25.
X.-Y. Liu, J. Kameda, J.W. Anderegg, S. Takaki, K. Abiko, and C.J. McMahon: Mater. Sci. Eng. A, vol. 492, 2008, pp. 218-20.
Q. Wu and M.A. Zikry: Materials Science and Engineering A, vol. 661, 2016 pp. 32-9.
Y. Ro, S.R. Agnew, and R.P. Gangloff: Metall. Mater. Trans. A, vol. 39, 2008, pp. 1449-65.
T. Watanabe and S. Tsurekawa: Mater. Sci. Eng. A, vol. 387–389, 2004, pp. 447-55.
D.H. Lassila and H.K. Birnbaum: Acta Metall., vol. 34, 1986, pp. 1237-43.
S. Kobayashi, S. Tsurekawa, T. Watanabe, and G. Palumbo: Scripta Mater., vol. 62, 2010, pp. 294-7.
C.A. Shuh, R.W. Minich and M. Kumar: Phil. Mag., vol. 83, 2003, pp. 711-26.
M. Fray and C.A. Shuh: Phil. Mag., vol. 85, 2005, pp. 1123-43.
H. Kitahara, R. Ueji, N. Tsuji, and Y. Minamino: Acta Mater., vol. 54, 2006, pp. 1279-88.
T. Furuhara, T. Shinyoshi, G. Miyamoto, J. Yamaguchi, N. Sugita, N. Kimura, N. Takemura, and T. Maki: ISIJ Intl., vol. 43, 2003, pp. 2028-37.
C.A. Apple and G. Krauss: Acta Metall., vol. 20, 1972, pp. 849-56.
K. Sato: Ph.D. Dissertation, University of California at Berkeley, Berkeley, CA, 2002.
P.M. Novotny: in Fundam. Aging Tempering Bainitic Martensitic Steel Prod., TMS-AIME, Warrendale, PA, 1993, pp. 215–36.
G. Wang, Y. Yan, J. Li, J. Huang, L. Qiao, and A.A. Volinsky: Matls. Sci. Engr. A, vol. 586, 2013, pp. 142-8.
J.P. Hirth: Metall. Trans. A, vol. 11, 1980, pp. 861-90.
G.L. Pioszak, Yongwon Lee, W.M. Garrison, and R.P. Gangloff: Effect of Liquid Nitrogen Chill on SCC of AerMet ® 100 Ultra-High Strength Steel, CorrDefense, NACE International, Houston, TX, 2012. (Available from the authors since https://www.corrdefense.org/External/ViewList.aspx?ListID=999 is incomplete.)
J.T. Burns, Z.D. Harris, J.D. Dolph, and R.P. Gangloff: Metall. Mater. Trans. A, vol. 47, 2016, pp. 990-7.
H. Huang and W.W. Gerberich: Acta Metall., vol. 42, 1994, pp. 639-47.
J. Weertman, I.-H Lin and R. Thomson, Acta Metall., vol. 42, 1983, pp. 473–82.
P. Doig and G.T. Jones: Metall. Trans. A, 1977, vol. 8, pp. 1993–98.
T.-Y. Zhang and J. E. Hack: Metall. Mater. Trans. A, vol. 30A, 1999, pp. 155-9.
D. Li, R. P. Gangloff, and J. R. Scully: Metall. Trans. A, vol. 35, 2004, pp. 849-64.
W.W. Gerberich, R.A. Oriani, M. J. Lii, X. Chen, and T. Foecke: Philos. Mag. A, vol. 63, 1991, pp. 363-76.
J.R. Scully and P.J. Moran: J. Electrochem. Soc., vol. 135, 1988, pp. 1337-48.
R. A. Oriani: Acta Metall., vol 18, 1970, pp. 147-57.
Y.D. Park, I.S. Maroef, A. Landau, and D.L. Olson: Weld. J., pp. 27S–35S, February, 2002.
H.J. Grabke and E. Rieche: Mater. Technol., vol. 34, 2000, pp. 331-42.
A. Brass, F. Guillon, and S. Vivet: Metall. Trans. A, vol. 35, 2004, pp. 1449-64.
A. Turnbull: Corros. Rev., vol. 30, 2012, pp. 1-17.
S.M. Al-Ghamdi: Ph.D. Dissertation, University of Virginia, Charlottesville, VA, 2010.
Acknowledgments
GLP was sponsored by the DoD Office of Corrosion Policy and Oversight through a funding agreement administered by the United States Air Force Academy. RPG was supported by Alcoa and Northrup Grumman Corporation Faculty Affiliate Programs. A portion of the crack growth experiments were conducted by J.K. Donald at Fracture Technology Associates, sponsored by Benet Laboratories on behalf of the US Army Contracting Command Joint Munitions & Lethality Contracting Center. Emilio Martinez-Pineda performed the crack tip analysis that yielded Figure 15. Discussions with J.R. Scully, W.M. Garrison, Jr., J. Sebastian, and G.B. Olson are gratefully acknowledged. The views and conclusions contained herein are those of the authors and should not be interpreted as necessarily representing the polices and endorsements, either expressed or implied, of the US Air Force Academy or the US Government.
Author information
Authors and Affiliations
Corresponding author
Additional information
Manuscript submitted October 22, 2016.
Rights and permissions
About this article
Cite this article
Pioszak, G.L., Gangloff, R.P. Hydrogen Environment Assisted Cracking of Modern Ultra-High Strength Martensitic Steels. Metall Mater Trans A 48, 4025–4045 (2017). https://doi.org/10.1007/s11661-017-4156-0
Received:
Published:
Issue Date:
DOI: https://doi.org/10.1007/s11661-017-4156-0