Abstract
Mitigating exotic species invasions requires reliable information on abundance and source-sink dynamics to evaluate effectiveness of control efforts. Despite being present in the southeastern United States for centuries, wild pigs (Sus scrofa) continue to invade new areas, including the Sam D. Hamilton Noxubee National Wildlife Refuge (NNWR) in Mississippi, a forested landscape with an emerging wild pig population. Considering the early stage of invasion, we designed and implemented a non-invasive hair sampling technique to estimate abundance and relatedness of wild pigs within the NNWR. Following 8-week sampling periods in 2021 (n = 160 samples) and 2022 (n = 159 samples), we identified 38 and 26 unique individuals, respectively, although recapture rates were too low to yield precise abundance estimates. We also identified low heterozygosity indicating high relatedness among our population which likely reflected lack of barrier features to gene flow and/or a limited number of external source populations. Finally, we observed positive trends in viable samples collected relative to sampling week, suggesting more optimal sampling period(s) may exist (e.g., autumn). We also acknowledge that use of grain in more accessible areas rather than our remote approach using scent-only attractants may increase hair snare attractiveness and wild pig encounters, while permitting increased sampling frequency. Collectively, these modifications (i.e., altered season, bait type, and access) provide viable pathways to increase precision for abundance estimates. When used concomitantly with ongoing monitoring and control efforts, non-invasive hair sampling will contribute to a more holistic understanding of this exotic species and better inform management actions.
Similar content being viewed by others
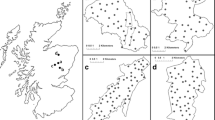
Avoid common mistakes on your manuscript.
Introduction
Understanding wildlife population dynamics equips natural resource managers and biologists with necessary information to effectively monitor changes in abundance and evaluate effectiveness of management or control strategies (Dolbeer 1998). Various active and passive methods have been used to estimate the abundance of wildlife populations. Active methods traditionally have included spotlight surveys (Collier et al. 2007), aerial surveys (Hone 2008), or capture-mark-recapture techniques, the latter of which have considerable utility in small mammal (Jung et al. 2020) and insect (Parmenter and MacMahon 1989) applications but can become laborious or otherwise risky when directly handling larger fauna. While passive methods have most commonly involved camera-trap surveys (Rovero et al. 2013), recent advances in DNA technology have enabled non-invasive genetic sampling which has shown promise in producing precise and accurate abundance estimates within a capture-recapture framework (Gardner et al. 2009). Genetic approaches allow for an examination of heterozygosity at unique loci (Nei 1978; Waits et al. 2001) and individual profiling through identification of specific genotypes and sex (Waits et al. 2001; Waits and Paetkau 2005). Combined with capture histories of individuals within a sample, abundance estimation within a capture-recapture framework becomes possible using information unobtainable by other passive techniques (Lukacs and Burnham 2005). Such passive methods may be especially useful when tasked with addressing exotic species invasions that require population monitoring, but in which post-capture release is undesirable or infeasible. Within areas occupied by exotic species, genetic investigations can allow for estimation of population size (Piggott et al. 2008), while also identifying source populations, population bottlenecks, and genetic variation (Tsutsui et al. 2000; Allendorf and Lundquist 2003; Zalewski et al. 2010), helping to inform natural resource managers and biologists on the magnitude of emerging invasions and effectiveness of control actions (e.g., selective removal, trapping).
Among genetic non-invasive sampling approaches, fecal DNA sampling has been most common and may be especially useful considering its randomness compared to samples obtained using attractants (King et al. 2021). However, sample degradation can occur rapidly due to high temperature, humidity, and precipitation levels, reducing amplification success and increasing genotyping errors (Taberlet and Luikart 1999; Broquet et al. 2007; Brinkman et al. 2010). While use of attractants within alternative passive sampling methods (e.g., barbed wire hair snares) may present other inherent concerns (e.g., sex- and age-related avoidance of sampling stations leading to capture bias; Wold and Wirsing 2020), the quality of DNA collected from hair samples tends to exceed fecal samples and has become the preferred collection method for many species (Depue and Ben-David 2007; Herr and Schley 2009; Murphy et al. 2016) but is still of less quality and quantity than required for genomic approaches such as RAD-seq (Andrews et al. 2016; Carroll et al. 2018).
The wild pig (Sus scrofa) is an exotic species (i.e., a cross between Eurasian wild boar and domestic swine) with invasion origins in the southeastern United States dating back to the 1500 s (Keiter et al. 2016; Lewis et al. 2019). This species has continued population expansions due to not only natural dispersal capabilities (Snow et al. 2017), but also anthropogenic support including translocation by hunters (Hernández et al. 2018). As wild pig distributions continue to expand and population growth goes largely unchecked in many areas due to lack of natural predators (i.e., Jeschke et al. 2012) and impactful anthropogenic control measures (e.g., aerial gunning; Davis et al. 2018; trapping; Gaskamp et al. 2021), ecologically sensitive areas, including those within the United States Fish and Wildlife Service’s National Wildlife Refuge System, continue to experience emerging invasions and require reliable means for estimating and monitoring wild pig population size.
Wild pig abundance has been measured using active (e.g., hunter surveys; Massei et al. 2015; trap removal models; Davis et al. 2020) and passive methods (e.g., track counts; Engeman et al. 2013; pellet counts; Ferretti et al. 2016; camera trapping; Massei et al. 2018; fecal DNA sampling; Davis et al. 2020). Among passive methods, camera trapping can yield wild pig abundance estimates consistent with estimates derived using other passive methods, although its effectiveness increases when used on populations with visually identifiable individuals (Schlichting et al. 2020). Furthermore, this method can become expensive and laborious due to the number of cameras required, with biases induced as cost reductions produce variability (Davis et al. 2020). Another passive method, genetic sampling, has shown promise in producing precise and accurate abundance estimates within a capture-recapture framework (Kierepka et al. 2016). Although use of genetic information in wild pig investigations has focused on fecal DNA collection (Ebert et al. 2012; Kierepka et al. 2016; Davis et al. 2020), issues with sample degradation can become especially problematic in the southeastern United States which experiences less than ideal environmental conditions (e.g., high temperature, humidity, and precipitation). Also, given the association between wild pigs and woody wetland (i.e., bottomland hardwood) areas, which may exacerbate these issues, development of a method that obtains high quality DNA samples from wild pigs for abundance estimation that does not use fecal samples is warranted. Hair DNA sampling for wild pigs remains seemingly novel despite its potential to estimate abundance and relatedness while improving understanding of wild pig invasions and better informing management decisions.
Our objectives were to (1) design and implement a hair DNA sampling technique, (2) estimate abundance of wild pigs in a landscape characterized by recent invasion, and (3) estimate genetic heterozygosity to examine relatedness relative to contributing source population(s). Non-invasive genetic sampling using hair snares, while relatively novel for wild pigs, could be an efficient tool for improving understanding of the current magnitude of wild pig invasions and better informing management decisions within areas which may make other sampling approaches (i.e., fecal DNA) less feasible. We expected that visitation of hair snares by wild pigs and estimates of abundance would relate to sex, age, and social group characteristics, with abundance estimates driven primarily by DNA sample collections from sounders (which are more philopatric and tend to exhibit greater site fidelity) relative to adult males (Keuling et al. 2008). Therefore, adult females and their family groups would contribute most viable hair samples. We also expected that the genetic structure of the sample population would be reflective of a highly related population due to its emerging status and lack of natural and anthropogenic landscape barriers, with low accompanying heterozygosity values among microsatellite loci.
Materials and methods
Study area
The Sam D. Hamilton Noxubee National Wildlife Refuge (hereafter, NNWR; Fig. 1) encompasses 19,425 ha in east-central Mississippi, USA with bottomland hardwoods (i.e., woody wetlands; 52.2%) and upland forests (i.e., evergreen, deciduous, and mixed; 40.4%) collectively dominating the landscape, while remaining land cover types (e.g., water, developed) comprise the remaining 7.4% (Dewitz 2019). In addition to the presence of wild pigs since 2014, native mammals include white-tailed deer (Odocoileus virginianus), raccoon (Procyon lotor), opossum (Didelphis virginiana), bobcat (Lynx rufus), and coyote (Canis latrans). Elevation ranges from 193 to 603 m, and the Noxubee River, Cypress Creek, Loakfoama Creek, and Oktoc Creek represent major perennial streams that cross the NNWR centrally from west to east. There are a limited number of county, refuge, and private roads (and one highway), and a few private residences representing development on the NNWR. Annual temperatures ranged 12.7–26.2 °C and monthly precipitation averaged 103.8 mm during this study (National Oceanic and Atmospheric Administration 2023).
Sampling sites
We began to implement wild pig hair snares by determining the wild pig distribution across the NNWR. During 2020–2021, we used wild pig damage transects (T. Evans, unpublished data) to identify areas (i.e., 1-km2 grid cells) with concentrations of rooting activity and/or wallows for deployment of hair snares (Fig. 1). Per Evans et al. In press, we used optimal non-grain attractants of used cooking oil (i.e., fish fryer grease) and orange marmalade (Great Value, Arkansas, USA) which adhered to area restrictions on grain deployment, supported hair snare activation in remote areas, and minimized hair snare visits by non-target species (e.g., raccoon). In 2021, an attractant was randomly assigned to each individual hair snare (i.e., both attractants used n = 2 times per cluster) and retained throughout the sampling period. In 2022, attractants were randomly assigned to hair snares but alternated weekly to encourage increased visitation through novelty. We replenished attractants weekly during hair sampling.
We designed and deployed 20 wild pig hair snares in 2021 based on our wild pig damage transects and the following methods (Fig. 1). We deployed hair snares in clusters (n = 5; Fig. 1) to prevent territorial individuals (e.g., adult males) from preventing other wild pigs from accessing hair snares and creating a demographic bias within our sample (Ebert et al. 2010). We randomly generated hair snare locations (n = 4) within each 1-km2 cell in ArcGIS (Environmental Systems Research Institute 2017) and spaced clusters > 2 km to promote independence between clusters and capture genetic heterozygosity across the NNWR. Average wild pig home range sizes among recent studies within the Southeast (Gaston et al. 2008; Friebel and Jodice 2009; Sparklin et al. 2009) suggest a 4-km minimum buffer for independence. However, we observed spatial clustering among collared pigs across the NNWR following known landscape features (e.g., rivers, creeks, roads, etc.) suggesting independence among clusters could be achieved with at least a 2 km buffer, which allowed for the inclusion of additional clusters. For each hair snare, two strands of barbed wire were stretched around trees (n = 2) and T-posts (n = 2) in a square formation with a perimeter of 32 m, and a non-grain attractant was placed in a decentralized manner on three tree trunks or stumps within the square. Barbed wire strands were placed 40–50 cm and 15–20 cm above ground level to obtain samples from adult and juvenile wild pigs, respectively (Fig. 2). However, lower strands were only used on two randomly assigned sides for each hair snare to reduce impediment of wire crossings by adults (Fig. 3).
Hair snare corral design used for genetic sampling of wild pigs (Sus scrofa) in the Sam D. Hamilton Noxubee National Wildlife Refuge (NNWR) in 2021–2022. Two sides were single upper stands and the other two sides were double-stranded, and although only trees are shown, a combination of two trees and two T-posts were used as corners for each hair snare
Hair sampling was conducted annually during 8 weeks from August–October, 2021–2022, with hair snares checked approximately weekly. However, during 2021, hair snares in two clusters (i.e., Roberts, Skinner; Fig. 1) became inundated during week 4 by Hurricane Ida, an event that prevented sampling of those hair snares (n = 8) and adversely impacted all clusters (see below). In 2022, all hair snares were sampled weekly. To evaluate hair snare effectiveness, we monitored wild pig contact and/or crossing events with barbed wire strands with a single remote camera trap (FORCE-20, Spypoint, Quebec, CA) during 2021. In 2022, however, remote cameras (n = 4) were placed on each side of hair snares (n = 7) with the greatest number of samples collected in 2021 to improve our understanding of wire contacts and/or crossings relative to resulting samples, as this was an issue identified in the only other study that tested hair snares for wild pigs (Ebert et al. 2010).
Sample collection, processing, and analysis
All hair present on an individual barb was collected and treated as a single sample (Fig. 3). Samples were collected using single-use latex gloves and sterilized tweezers. We sterilized all sampling utensils and barbs between sample collections using a butane torch. All samples were stored in coin envelopes with desiccant at room temperature to prevent sample degradation. We labeled each sample with date, hair snare identification, side of collection, and barb number on that side.
Hair samples were genotyped by Wildlife Genetics International Incorporated (WEGI; British Columbia, CAN). They purified DNA from samples using QIAGEN® DNeasy Blood and Tissue Kits (Germantown, Maryland, USA), by following the manufacturer’s protocol and using < 10 clipped guard hair roots supplemented by up to 30 whole underfur hairs depending on guard hair availability. They evaluated published primers for 20 microsatellite loci for variability, sensitivity and specificity (see Online Resource 1; Robic et al. 1995, Rohrer et al. 1994, Alexander et al. 1996a, 1996b, Krause et al. 2008, Ellegren et al. 1993). All initial multilocus genotyping efforts and evaluations of heterozygosity were conducted using trapped (n = 18 individuals) and hair snare-sampled individuals (see Results) from the NNWR during 2020–2021. A set of the 8 most variable loci was expected to have a match probability low enough to accurately identify individuals (Table 1). Across these microsatellite loci, observed alleles, observed heterozygosity, and expected heterozygosity suggested low levels of genetic diversity within the NNWR wild pig population (Table 1). Primers were also developed by WGI for a sex marker using porcine ZFX and ZFY sequences downloaded from Genbank (Accession numbers DQ179231 and FP102717). These primers were validated using samples from collared and non-collared individuals (n = 9 males, n = 9 females) trapped during GPS collar deployments in the NNWR from 2020–2021 (T. Evans, unpublished data).
Each sample from both years of hair sampling was analyzed for individual identity using the above marker set (8 microsatellites plus ZFX/ZFY for sex). Samples with incomplete 9-locus genotypes were culled due to higher match probabilities and error rates. To prevent identification of false individuals, error-checking involved the reanalysis of mismatching markers in pairs of genotypes similar enough to have possibly arisen from genotyping errors (Paetkau 2003; Kendall et al. 2009). Only individuals identified from hair samples and not trapped pigs were used during abundance estimation. Additional information regarding PCR thermocycling can be found in Online Resource 2.
Final genotypes were used to estimate abundance in the NNWR with conditional likelihood closed-capture models (Huggins 1989) in Program MARK v.10.1 (White and Burnham 1999). We used Akaike’s Information Criterion corrected for small sample size (AICc; Akaike 1973; Sugiura 1978) within an information theoretic approach to evaluate a candidate set of models that treated capture probabilities as constant or allowed them to vary by additive effects of week and year, or as interactions between these terms. We also considered a linear trend on capture probabilities in 2022 based on apparent weekly increases in the number of viable samples collected. Weekly precipitation was also a factor of interest but could not be investigated due to the staggered nature of sampling within each sampling week (i.e., hair snare clusters sampled on different days each week due to labor involved, thereby leading to differential weekly precipitation totals). However, we accounted for known flooding effects of Hurricane Ida (i.e., 142.8 mm of rainfall; National Oceanic and Atmospheric Administration 2023) in week 4 of 2021 by fixing its capture probability to zero in all models. We estimated overdispersion for the global model using Fletcher \(\widehat{c}\) (Fletcher 2012) and used model averaging for derived estimates of abundance (Stanley and Burnham 1998).
Results
In 2021, we obtained 159 hair samples (with used cooking oil: n = 88; orange marmalade: n = 71), of which 49 samples (30.8%) were viable and yielded individual genotypes. There were 38 individuals (n = 20 males, n = 18 females) among these samples, with 31 individuals represented by a single sample each and 7 individuals detected among 2–5 samples. However, among these there were only 5 recaptures (i.e., individuals detected > 1 sampling week; Table 2). In 2022, we obtained 160 samples (with used cooking oil: n = 92; orange marmalade: n = 68), of which 42 samples (26.3%) were viable and yielded individual genotypes. There were 26 individuals (n = 15 males, n = 11 females) among these samples, with 16 individuals represented by a single sample each and 10 individuals detected among 2–5 samples. Recaptures were rare, with only 3 individuals detected during > 1 sampling week (Table 3), and no individuals captured in 2021 were recaptured in 2022. We identified noticeable trends in sample collections in both years, with 65.3% (32/49) and 71.4% (30/42) of all viable samples collected in weeks 6–8 in 2021 and 2022, respectively (Online Resources3, 4).
Among camera-monitored hair snares in 2022, we documented 90 wire contacts and/or crossing events, of which 52 (57.8%) yielded accompanying samples for individual, age, and sex identification. Among all hair snares, we identified noticeable disparities between samples yielded from our top strands (2021: n = 145; 2022: n = 144) relative to our bottom strands (2021: n = 14; 2022: n = 16). Further, although we could only account for additive, interactive, and observed effects of sampling week and year in our candidate set of models, we identified differences in weekly precipitation totals during weeks that yielded viable samples relative to those that did not across individual wild pigs sampled, although these differences were more apparent in 2021 (weeks with viable samples: 33.5 ± 3.9 mm; weeks without viable samples: 51.9 ± 2.9 mm) than 2022 (weeks with viable samples: 19.9 ± 4.9 mm; weeks without viable samples: 23.8 ± 2.1 mm; National Oceanic and Atmospheric Administration 2023).
We detected no overdispersion using Fletcher \(\widehat{c}\). Our top-ranked Huggins closed-capture model, in which capture probabilities were treated as equal in all weeks except for week 4 (2021) and as a linear trend by week (2022), garnered 87.4% of model weights, with no other candidate model receiving > 7.5% of remaining model weights (Tables 3, 4). Model averaged estimates of abundance for 2021 (n̂ = 148; 95% CI = 80–331) and 2022 (n̂ = 107; 95% CI = 50–293) were accompanied by high variability with coefficients of variation of 0.40 and 0.52, respectively, which reflected imprecision (relative to desired coefficients of variation < 0.2; Pollock et al. 1990).
Discussion
Visitation rates did not appear to be biased by philopatry of female-led sounders, contrasting our prediction but also appearing to avoid a related concern in the only other study that investigated feasibility of hair snare use for wild pigs (Ebert et al. 2010). While our basis for this conclusion relates to the apparent evenness of sampling by sex in our study, it would also be plausible to attribute this finding to sampling of juvenile males within sounders rather than adult males. However, it is notable that, of the 17 males observed with camera monitoring of wire strands during our second year of study, fourteen were solitary adult males, an additional adult male with a sounder, and two subadult males in the same sounder which suggests juvenile males did not bias our sex ratio. Meanwhile, our wire strand design was biased towards subadult and adult age classes or at least did not snare hair from piglets. Despite our limited information on sounder use from genetic sample frequency and restricted field-of-view camera monitoring of select strands during 2022, only 12 wire contacts (23%) occurred when multiple pigs were in and around the hair snare suggesting a sounder group (i.e., multiple subadults, adults with younger pigs, piglets).
Surprisingly, no individuals captured in 2021 were recaptured in 2022, an unexpected finding since hair snare locations were the same during both years. This may reflect an association of hair snares with minimal nutritional benefit (i.e., scent only) given prior experiences of wild pigs with these locations. Alternatively, both seasonal and flash flooding of the NNWR leads to displacement of wild pigs from areas within which many of our hair snares were located, and upon recession of flood waters, wild pigs may have relocated to other areas within or adjacent to the NNWR, especially given the contiguous properties of the NNWR’s woody wetlands and ability of these areas to meet foraging and thermoregulatory requirements. However, failure to detect an individual from 2021 at any hair snare cluster in 2022, indicates that cluster placement and number, while important to our assumption of cluster independence within a single sampling year, may not be optimal for places like bottomland hardwoods in the southeastern U.S., whose flooding propensity likely displaces wild pigs to great extents across the landscape between years.
We identified notable trends in sample collection both years, with most viable samples collected during the final three weeks. While we found a positive linear trend in viable samples by week in 2022, this relationship was less discernible in 2021 and may have been related to the effects of Hurricane Ida during the intermediate stages of our sampling effort. Regardless, the similarly high percentages of viable samples collected during the final weeks in each year suggests that an optimal window for sampling exists for the NNWR, and we may have merely sampled during its commencement (i.e., during our final weeks). While possible explanations for this phenomenon could include increases in hair snare attractiveness due to accumulating effects of scent deployment or higher sample quality due to a temporal effect (i.e., more recently collected samples), we believe that these explanations are less likely to represent the observed trend, especially considering the viability of hair samples collected from trapped wild pigs (i.e., 18/18, or 100% genotyping success) which were stored in an identical manner to hair snare samples and for longer durations. Rather, temporal shifts in space use relative to increases in hard mast availability (Froehly et al. 2020) or increasing anthropogenic pressures (i.e., hunting-related; Scillitani et al. 2010) near the end of the sampling period may have led to greater numbers of individuals in remote areas within which hair snares were located. Trends could also be related to differential weekly precipitation, which is demonstrated through contrasts between successful and unsuccessful sample collections from unique individuals by week, with this pattern being more discernible in 2021 than 2022, the latter of which was comprised by generally dry conditions both preceding and during the sampling period. Furthermore, decreasing ambient temperatures from August to October, could have helped increase sample viability (Piggott 2004; Murphy et al. 2007). Our inability to detect 2021 captures in 2022 could also potentially be attributed to these conditions, given differences observed at multiple hair snare clusters between 2021 and 2022 (i.e., moist conditions vs. drought conditions, respectively) which may have led to displacement of wild pigs in a manner comparable to flooding at the opposite extreme. Regardless of the factor or factors which yielded the consistent pattern we observed in our collection of viable samples across years, this will present an issue for not only the NNWR but other public lands which must impose baiting restrictions at a time (i.e., October 1, or the start of archery season) during which wild pigs may be most responsive to attractants, whether non-grain or grain. The low overall number of viable samples, while concerning, may also reflect the emergent status of wild pigs in the NNWR, and while our method would conceivably benefit from continued population growth, this represents a suboptimal tradeoff which will likely be accompanied by continued degradation of natural ecosystems within the NNWR.
Although the low number of individuals captured and recaptured across sampling years was concerning, our method still showed utility and potential to yield samples needed for reliable abundance estimates. For example, wire contact and/or crossing rates during our 2022 camera monitoring of hair snares were more than twice the previously reported rates (14–25%; Ebert et al. 2010), especially considering these percentages across studies represent total samples collected without regarding processing. While Ebert et al. (2010) also cited issues with behavioral biases (i.e., dominant individuals excluding subdominants) which led to unequal capture probabilities, our study appeared to remedy this particular issue, although this was not explicitly tested and other observable and latent heterogeneities are also known to undermine underlying assumptions of equal capture probability (Otis et al. 1978). Further, our study still identified an issue with sampling juveniles via the bottom strand, as evidenced by the low number of samples yielded across sampling years. Use of bottom stranding on only two sides of each hair snare may have contributed to this disparity, with juveniles simply gaining entry to the attractant through a top strand-only side; however, this design was deliberately implemented to prevent issues in impeding wire contacts and/or crossing events by adults. While the top strand appeared to be effective in sampling adults, identifying optimal strand heights may present a challenge in the southeastern United States and other areas where wild pig breeding is asynchronous and leads to a broad spectrum of possible wild pig sizes (i.e., heights) at any given time. Because of this, the use of double stranding on all sides with a more effective attractant (i.e., grain-scent combination) may remedy our concerns of impeding adult visitation. Alternatively, our method as currently designed may be limited to estimation of the breeding population (i.e., adults).
Even when we alternated non-grain attractants on a weekly basis in 2022 to encourage repeat visitation, we failed to increase recaptures, an issue that yielded coefficients of variation which indicated imprecision in abundance estimates. We also encountered difficulties in obtaining samples generally regarded as high quality for genotyping success (i.e., ≥ 3 guard hairs with roots; D. Paetkau, pers. Comm.), with few of our samples (i.e., 10.7% and 16.3% in 2021 and 2022, respectively) meeting this criterion, suggesting the passive nature of our method may become problematic when less enticing attractants are deployed. To remedy this issue, we cautiously encourage use of grain-based attractants while still complementing grain with non-grain attractants (e.g., orange flavor) to improve association of hair snares with nutritional (i.e., caloric) benefit and to enhance attractiveness, respectively. Given the ability of wild pigs to rapidly deplete grain, an increase in sampling frequency and bait replenishment (e.g., twice weekly) would be afforded by distributing hair snares closer to roads and trails and may collectively improve success in obtaining samples of higher quantity and quality (i.e., greater number of hairs/sample that were not exposed to environmental conditions long enough to degrade). This improvement, in turn, could plausibly yield the numbers of captures and recaptures needed to generate more precise abundance estimates with more complex spatial capture-recapture models incorporating factors affecting individual capture heterogeneity (e.g., differential space use and detection probabilities between sexes; see Efford and Mowat 2014). While these modifications were not feasible in our study, which required use of non-grain attractants in remote or otherwise inaccessible areas for grain deployment, these next steps appear to be warranted and should maximize the usefulness of this technique. Additionally, while we placed clusters in a manner that increased the chance of sampling independence between detections, some studies do show that a greater density of traps will yield less biased estimates particularly in social species, as long as they are randomly placed (e.g., Gupta et al. 2017), and a greater density of snares certainly can increase overall sample size, something that would be attempted in future efforts.
Consistent with our prediction, the wild pig population had low heterozygosity and genetic diversity (i.e., < 0.70; Paetkau 2003) compared to other species in DNA inventories (Paetkau 2003) and European wild boar and domestic pig populations (Šprem et al. 2014). However, compared to another wild pig population in the Southeast, observed and expected heterozygosity values averaging 0.64 and 0.65, respectively, are common (Kierepka et al. 2016). Kierepka et al. (2016) developed microsatellite markers from wild pig fecal DNA among 40 culled wild pigs at a Savannah River Site (SRS) in South Carolina, USA. Unlike our NNWR population, the SRS wild pig population has been established since at least the 1950s (Mayer and Brisbin 1991; Beasley et al. 2014). Hence, a founder effect from a recent invasion as with the NNWR may also persist in older populations. Wild pigs that represent the primary source of the NNWR invasion are believed to be those which gradually dispersed to the NNWR from one or more nearby properties that introduced wild pigs in the 1980s. Given the apparent attractiveness of the NNWR to wild pigs through its capacity to meet foraging (e.g., moist, easy-to-root soils) and thermoregulatory requirements (i.e., majority of the NNWR is woody wetlands containing a network of perennial streams which may act as corridors), increases in genetic variation may eventually be possible if wild pigs immigrate into the NNWR from other nearby source populations connected via such corridor features. The lack of substantive barrier features, whether anthropogenic (e.g., major highways, residential development) or natural (e.g., rugged terrain, large rivers), may facilitate such future invasions, and lack of substantive lethal pressures imposed on wild pigs in the NNWR (i.e., incidental take of ~ 100 wild pigs/year during native game hunting seasons; T. Carpenter, United States Fish and Wildlife Service, pers. Comm.) may further exacerbate this issue. Whereas, frequently hunted populations or those constrained by trapping, like SRS, may experience less potential of new gene flow from pigs avoiding these areas causing genetic diversity to remain relatively low.
Considering the successes relating to our design and implementation of this novel technique for wild pigs which include improvements in sample-yielding wire contacts and crossings, insights into optimal sampling timing, and reduction in behavioral biases, we encourage continued use of this method, especially considering these additional minor modifications may yield reliable abundance estimates within the sampling area and allow for extrapolation to a broader scale given other information, such as occurrence, which may be obtained using other non-invasive techniques (e.g., damage transects; T. Evans, unpublished data). Ultimately, these steps will allow natural resource managers and biologists to monitor population abundance changes through time, information which may prove especially useful when used concomitantly with coordinated control efforts.
Data availability
Data is available through Mississippi State University’s Scholar Junction (Evans et al. 2024).
References
Akaike H (1973) Information theory and an extension of the maximum likelihood principle. In Petrov BN, Csaki F (eds) Proceeding of the Second International Symposium on Information Theory, Akademiai, Kiado, Budapest, Hungary. pp 267–281
Alexander LJ, Rohrer GA, Beattie CW (1996a) Cloning and characterization of 414 polymorphic porcine microsatellites. Anim Genet 27(3):137–148. https://doi.org/10.1111/j.1365-2052.1996.tb00941.x
Alexander LJ, Troyer DL, Rohrer GA, Smith TPL, Schook LB, Beattie CW (1996b) Physical assignments of 68 porcine cosmid and lambda clones containing polymorphic microsatellites. Mamm Genome 7:368–372. https://doi.org/10.1007/s003359900106
Allendorf FW, Lundquist LL (2003) Introduction: population biology, evolution, and control of invasive species. Conserv Biol 1:24–30
Andrews KR, Good JM, Miller MR, Luikart G, Hohenlohe PA (2016) Harnessing the power of RADseq for ecological and evolutionary genomics. Nature Rev Genet 17(2):81–92. https://doi.org/10.1038/nrg.2015.28
Beasley JC, Grazia TE, Johns PE, Mayer JJ (2014) Habitats associated with vehicle collisions with wild pigs. Wildl Res 40:656–660. https://doi.org/10.1071/WR13061
Brinkman TJ, Schwartz MK, Person DK, Pilgrim KL, Hundertmark KJ (2010) Effects of time and rainfall on PCR success using DNA extracted from deer fecal pellets. Conserv Genet 11:1547–1552. https://doi.org/10.1007/s10592-009-9928-7
Broquet T, Menard N, Petit E (2007) Noninvasive population genetics: a review of sample source, diet, fragment length and microsatellite motif effects on amplification success and genotyping error rates. Conserv Genet 8:249–260. https://doi.org/10.1007/s10592-006-9146-5
Carroll EL, Bruford MW, DeWoody JA, Leroy G, Strand A, Waits L, Jinliang W (2018) Genetic and genomic monitoring with minimally invasive sampling methods. Evol Appl 11:1094–1119. https://doi.org/10.1111/eva.12600
Collier BA, Ditchkoff SS, Raglin JB, Smith JM (2007) Detection probability and sources of variation in white-tailed deer spotlight surveys. J Wildl Manage 71(1):277–281. https://doi.org/10.2193/2005-728
Davis AJ, Leland B, Bodenchuk M, VerCauteren KC, Pepin KM (2018) Costs and effectiveness of damage management of an overabundant species (Sus scrofa) using aerial gunning. Wildl Res 45(8):696–705. https://doi.org/10.1071/WR17170
Davis AJ, Keiter DA, Kierepka EM, Slootmaker C, Piaggio AJ, Beasley JC, Pepin KM (2020) A comparison of cost and quality of three methods for estimating density for wild pig (Sus scrofa). Sci Rep 10:2047. https://doi.org/10.1038/s41598-020-58937-0
Depue JE, Ben-David M (2007) Hair sampling techniques for river otters. J Wildl Manag 71(2):671–674. https://doi.org/10.2193/2005-712
Dewitz J (2019) National land cover database (NLCD) 2016 Products: U.S. Geological Survey data release. https://doi.org/10.5066/P96HHBIE
Dolbeer RA (1998) Population dynamics: the foundation of wildlife damage management for the 21st century. Proc Vertebr Pest Conf 18:18. https://doi.org/10.5070/V418110312
Ebert C, Huckschlag D, Schulz HK, Hohmann U (2010) Can hair traps sample wild boar (Sus scrofa) randomly for the purpose of non-invasive population estimation? Eur J Wildl Res 56:583–590. https://doi.org/10.1007/s10344-009-0351-7
Ebert C, Knauer F, Spielberger B, Thiele B, Hohmann U (2012) Estimating wild boar Sus scrofa population size using faecal DNA and capture-recapture modelling. Wildlife Biol 18(2):142–152. https://doi.org/10.2981/11-002
Efford MG, Mowat G (2014) Compensatory heterogeneity in spatially explicit capture-recapture data. Ecology 95(5):1341–1348
Ellegren H, Johansson M, Chowdhary BP, Marklund S, Ruyter D, Marklund L, Bräuner-Nelson P, Edfors-Lilja I, Gustavsson I, Juneja RK, Andersson L (1993) Assignment of 20 microsatellite markers to the Porcine linkage map. Genomics 16(2):431–439. https://doi.org/10.1006/geno.1993.1207
Engeman RM, Massei G, Sage M, Gentle MN (2013) Monitoring wild pig populations: a review of methods. Environ Sci Pollut Res 20:8077–8091. https://doi.org/10.1007/s11356-013-2002-5
Environmental Systems Research Institute (2017) ArcGIS Desktop: Release 10.6.1. Redlands, CA: Environmental Systems Research Institute
Evans TE, Hileman ET, Boudreau MR, Strickland BK, Iglay RB (2024) Capture history data from hair snare sampling of wild pigs in Mississippi. College of Forest Resources Publications and Scholarship 26. https://doi.org/10.54718/KXKA1060
Evans TE, Boudreau MR, Fleming OR, Strickland BK, Street GM, Iglay RB (In press) No corn, no problem: a test for the best non-grain attractant for wild pigs. J SEAFWA
Ferretti F, Fattorini L, Sforzi A, Pisani C (2016) The use of faeces counts to estimate relative densities of wild boar in a Mediterranean area. Popul Ecol 58:329–334. https://doi.org/10.1007/s10144-016-0536-3
Fletcher DJ (2012) Estimating overdispersion when fitting a generalized linear model to sparse data. Biometrika 99:230–237. https://doi.org/10.1093/biomet/asr083
Friebel BA, Jodice PG (2009) Home range and habitat use of feral hogs in Congaree National Park South Carolina. Hum-Wildl Confl 3(1):49–63. https://doi.org/10.26077/2466-1s92
Froehly JL, Beane NR, Evans DE, Cagle KE, Jachowski DS (2020) Using multi-scale behavioral investigations to inform wild pig (Sus scrofa) population management. PLoS ONE 15(2):e0228705. https://doi.org/10.1371/journal.pone.0228705
Gardner B, Royle JA, Wegan MT (2009) Hierarchical models for estimating density from DNA mark–recapture studies. Ecol 90(4):1106–1115. https://doi.org/10.1890/07-2112.1
Gaskamp JA, Gee KL, Campbell TA, Silvy NJ, Webb SL (2021) Effectiveness and efficiency of corral traps, drop nets and suspended traps for capturing wild pigs (Sus scrofa). Animals 11(6):1565. https://doi.org/10.3390/ani11061565
Gaston W, Armstrong JB, Arjo W, Stribling HL (2008) Home range and habitat use of feral pigs (Sus scrofa) on Lowndes County WMA, Alabama. National Conference on Feral Hogs, St. Louis, Missouri, USA
Gupta M, Joshi A, Vidya TNC (2017) Effects of social organization, trap arrangement and density, sampling scale, and population density on bias in population size estimation using some common mark-recapture estimators. PLoS ONE 12(3):e0173609. https://doi.org/10.1371/journal.pone.0173609
Hernández FA, Parker BM, Pylant CL, Smyser TJ, Piaggio AJ, Lance SL, Milleson MP, Austin JD, Wisely SM (2018) Invasion ecology of wild pigs (Sus scrofa) in Florida, USA: the role of humans in the expansion and colonization of an invasive wild ungulate. Biol Invasions 20:1865–1880. https://doi.org/10.1007/s10530-018-1667-6
Herr J, Schley L (2009) Barbed wire hair traps as a tool for remotely collecting hair samples from beavers (Castor sp.). Lutra 52(2):123–127
Hone J (2008) On bias, precision and accuracy in wildlife aerial surveys. Wildl Res 35(4):253–257. https://doi.org/10.1071/WR07144
Huggins R (1989) On the statistical analysis of capture experiments. Biometrika 76(1):133–140. https://doi.org/10.1093/biomet/76.1.133
Jeschke JM, Aparicio LG, Haider S, Heger T, Lortie CJ, Pysek P, Strayer DL (2012) Support for major hypotheses in invasion biology is uneven and declining. NeoBiota 14:1–20. https://doi.org/10.3897/neobiota.14.3435
Jung TS, Boonstra R, Krebs CJ (2020) Mark my words: experts’ choice of marking methods used in capture–mark–recapture studies of small mammals. J Mammal 101(1):307–317. https://doi.org/10.1093/jmammal/gyz188
Keiter DA, Mayer JJ, Beasley JC (2016) What is in a “Common” name? a call for consistent terminology for nonnative Sus scrofa. Wildl Soc Bull 40(2):384–387. https://doi.org/10.1008/wsb.649
Kendall KC, Stetz JB, Boulanger J, Macleod AC, Paetkau D, White GC (2009) Demography and genetic structure of a recovering grizzly bear population. J Wildl Manag 73(1):3–17. https://doi.org/10.2193/2008-330
Keuling O, Stier N, Roth M (2008) Annual and seasonal space use of different age classes of female wild boar Sus scrofa L. Eur J Wildl Res 54:403–412. https://doi.org/10.1007/s10344-007-0157-4
Kierepka EM, Unger SD, Keiter DA, Beasley JC, Rhodes OE Jr, Cunningham FL, Piaggio AJ (2016) Identification of robust microsatellite markers for wild pig fecal DNA. J Wildl Manag 80(6):1120–1128. https://doi.org/10.1002/jwmg.21102
King SR, Schoenecker KA, Fike JA, Oyler-McCance SJ (2021) Feral horse space use and genetic characteristics from fecal DNA. J Wildl Manag 85(6):1074–1083. https://doi.org/10.1002/jwmg.21974
Krause E, Morrison L, Reed M, Alexander LJ (2008) Radiation hybrid mapping of 273 previously unreported porcine microsatellites. Anim Genet 33(5):477–485. https://doi.org/10.1046/j.1365-2052.2002.00938_9.x
Lewis JS, Corn JL, Mayer JJ, Jordan TR, Farnsworth ML, Burdett CL, VerCauteren KC, Sweeney SJ, Miller RS (2019) Historical, current, and potential population size estimates of invasive wild pigs (Sus scrofa) in the United States. Biol Invasions 21:2373–2384. https://doi.org/10.1007/s10530-019-01983-1
Lukacs PM, Burnham KP (2005) Review of capture–recapture methods applicable to noninvasive genetic sampling. Mol Ecol 14(13):3909–3919. https://doi.org/10.1111/j.1365-294X.2005.02717.x
Massei G, Kindberg J, Licoppe A, Gacic D, Sprem N, Kamler J, Baubet E, Hohmann U, Monaco A, Ozolins J, Cellina S, Podgorski T, Fonseca C, Markov N, Pokorny B, Rosell C, Nahlik A (2015) Wild boar populations up, numbers of hunters down? a review of trends and implications for Europe. Pest Manag Sci 71(4):492–500. https://doi.org/10.1002/ps.3965
Massei G, Coats J, Lambert MS, Pietravalle S, Gill R, Cowan D (2018) Camera traps and activity signs to estimate wild boar density and derive abundance indices. Pest Manag Sci 74(4):853–860. https://doi.org/10.1002/ps.4763
Mayer JJ, Brisbin IL Jr (1991) Wild pigs in the United States: their history, comparative morphology, and current status. University Georgia Press, Georgia
Murphy MA, Kendall KC, Robinson A, Waits LP (2007) The impact of time and field conditions on brown bear (Ursus arctos) faecal DNA amplification. Conserv Genet 8:1219–1224. https://doi.org/10.1007/sl10592-006-9264-0
Murphy SM, Cox JJ, Augustine BC, Hast JT, Guthrie JM, Wright J, McDermott J, Maehr SC, Plaxico JH (2016) Characterizing recolonization by a reintroduced bear population using genetic spatial capture–recapture. J Wildl Manag 80(8):1390–1407. https://doi.org/10.1002/jwmg.21144
National Oceanic and Atmospheric Administration (2023) Climate Data Online: Mississippi State University, US (USC00228374). <https://www.ncei.noaa.gov/cdo-web/>. Accessed 26 April 2023
Nei M (1978) Estimation of average heterozygosity and genetic distance from a small number of individuals. Genetics 89(3):583–590. https://doi.org/10.1093/genetics/89.3.583
Otis DL, Burnham KP, White GC, Anderson DR (1978) Statistical inference from capture data on closed animal populations. Wildl Monogr 62:3–135
Paetkau D (2003) An empirical exploration of data quality in DNA-based population inventories. Mol Ecol 12(6):1375–1387. https://doi.org/10.1046/j.1365-294X.2003.01820.x
Parmenter RR, MacMahon JA (1989) Animal density estimation using a trapping web design: field validation experiments. Ecology 70(1):169–179. https://doi.org/10.2307/1938424
Piggott MP (2004) Effect of sample age and season of collection on the reliability of microsatellite genotyping of faecal DNA. Wildl Res 31:485–493. https://doi.org/10.1071/WR03096
Piggott MP, Wilson R, Banks SC, Marks CA, Gigliotti F, Taylor AC (2008) Evaluating exotic predator control programs using non-invasive genetic tagging. Wildl Res 35(7):617–624. https://doi.org/10.1071/WR08040
Pollock KH, Nichols JD, Brownie C, Hines JE (1990) Statistical inference for capture-recapture experiments. Wildl Monogr 1:3–97
Robic A, Parrou J-L, Goureau A, Dalens M, Milan D, Gellin J (1995) Pig microsatellites isolated from cosmids revealing polymorphism and localized on chromosomes. Anim Genet 26(1):1–6. https://doi.org/10.1111/j.1365-2052.1995.tb02611.x
Rohrer GA, Alexander LJ, Keele JW, Smith TP, Beattie CW (1994) A microsatellite linkage map of the porcine genome. Genet 136(1):231–245. https://doi.org/10.1093/genetics/136.1.231
Rovero F, Zimmermann F, Berzi D, Meek P (2013) Which camera trap type and how many do I need? a review of camera features and study designs for a range of wildlife research applications. Hystrix 24(2):148–156. https://doi.org/10.4404/hystrix-24.2-8789
Schlichting PE, Beasley JC, Boughton RK, Davis AJ, Pepin KM, Glow MP, Snow NP, Miller RS, VerCauteren KC, Lewis JS (2020) A rapid population assessment method for wild pigs using baited cameras at 3 study sites. Wildl Soc Bull 44(2):372–382. https://doi.org/10.1002/wsb.1075
Scillitani L, Monaco A, Toso S (2010) Do intensive drive hunts affect wild boar (Sus scrofa) spatial behaviour in Italy? some evidences and management implications. Eur J Wildl Res 56:307–318. https://doi.org/10.1007/s10344-009-0314-z
Snow NP, Jarzyna MA, VerCauteren KC (2017) Interpreting and predicting the spread of invasive wild pigs. J Appl Ecol 54:2022–2032. https://doi.org/10.1111/1365-2664.12866
Sparklin BD, Mitchell MS, Hanson LB, Jolley DB, Ditchkoff SS (2009) Territoriality of feral pigs in a highly persecuted population on Fort Benning. Georgia J Wildl Manage 73(4):497–502. https://doi.org/10.2193/2007-585
Šprem N, Salajpal K, Safner T, Ðikic D, Jurić J, Curik I, Ðikic M, Cubric-Curik V (2014) Genetic analysis of hybridization between domesticated endangered pig breeds and wild boar. Livest Sci 162:1–4. https://doi.org/10.1016/j.livsci.2013.12.010
Stanley TR, Burnham KP (1998) Information-theoretic model selection and model averaging for closed-population capture-recapture studies. Biom J 40:475–494
Sugiura N (1978) Further analysis of the data by Akaike’s information criterion and the finite corrections: further analysis of the data by Akaike’s. Commun Stat Theory Methods 7(1):13–26. https://doi.org/10.1080/03610927808827599
Taberlet P, Luikart G (1999) Non-invasive genetic sampling and individual identification. Biol J Linn 68:41–55. https://doi.org/10.1111/j.1095-8312.1999.tb01157.x
Tsutsui ND, Suarez AV, Holway DA, Case TJ (2000) Reduced genetic variation and the success of an invasive species. Proc Natl Acad Sci USA 97(11):5948–5953. https://doi.org/10.1073/pnas.100110397
Waits LP, Paetkau D (2005) Noninvasive genetic sampling tools for wildlife biologists: a review of applications and recommendations for accurate data collection. J Wildl Manag 69(4):1419–1433. https://doi.org/10.2193/0022-541X(2005)69[1419:NGSTFW]2.0.CO;2
Waits LP, Luikart G, Taberlet P (2001) Estimating the probability of identity among genotypes in natural populations: cautions and guidelines. Mol Ecol 10(1):249–256. https://doi.org/10.1046/j.1365-294X.2001.01185.x
White GC, Burnham KP (1999) Program MARK: Survival estimation from populations of marked animals. Bird Study 46:S120–S139. https://doi.org/10.1080/00063659909477239
Wold K, Wirsing AJ, Quinn TP (2020) Do brown bears Ursus arctos avoid barbed wires deployed to obtain hair samples? A Videogr Assess Wildl Biol 2020(1):1–6. https://doi.org/10.2981/wlb.00664
Zalewski A, Michalska-Parda A, Bartoszewicz M, Kozakiewicz M, Brzezinski M (2010) Multiple introductions determine the genetic structure of an invasive species population: American mink Neovison vison in Poland. Biol Conserv 143(6):1355–1363. https://doi.org/10.1016/j.biocon.2010.03.009
Acknowledgements
The authors would like to express their gratitude to the United States Fish and Wildlife Service personnel at the Sam D. Hamilton Noxubee National Wildlife Refuge for their support, and to the reviewers for their constructive feedback. This research was funded by, and this publication is a contribution of, Mississippi State University’s Forest and Wildlife Research Center [McIntire-Stennis Project MISZ-085160] and Mississippi Agriculture and Forestry Experiment Station [HATCH Project MIS-085180].
Funding
This research was funded by, and this publication is a contribution of, Mississippi State University’s Forest and Wildlife Research Center [McIntire-Stennis Project MISZ-085160] and Mississippi Agriculture and Forestry Experiment Station [HATCH Project MIS-085180].
Author information
Authors and Affiliations
Contributions
Our study brings together authors from multiple countries, and all authors were engaged with the research and study design to ensure that diverse perspectives were considered. All authors conceived the ideas and designed methodology; TE collected the data; TE and EH analyzed the data; TE and RI led the writing of the manuscript. All authors contributed critically to the drafts and gave final approval for publication.
Corresponding author
Ethics declarations
Competing interest
The authors have no relevant financial or non-financial interests to disclose.
Additional information
Publisher's Note
Springer Nature remains neutral with regard to jurisdictional claims in published maps and institutional affiliations.
Supplementary Information
Below is the link to the electronic supplementary material.
Rights and permissions
Open Access This article is licensed under a Creative Commons Attribution 4.0 International License, which permits use, sharing, adaptation, distribution and reproduction in any medium or format, as long as you give appropriate credit to the original author(s) and the source, provide a link to the Creative Commons licence, and indicate if changes were made. The images or other third party material in this article are included in the article's Creative Commons licence, unless indicated otherwise in a credit line to the material. If material is not included in the article's Creative Commons licence and your intended use is not permitted by statutory regulation or exceeds the permitted use, you will need to obtain permission directly from the copyright holder. To view a copy of this licence, visit http://creativecommons.org/licenses/by/4.0/.
About this article
Cite this article
Evans, T.S., Hileman, E.T., Boudreau, M.R. et al. Can hair snares provide a reliable method for estimating abundance of an exotic ungulate?. Biol Invasions 26, 3017–3029 (2024). https://doi.org/10.1007/s10530-024-03363-w
Received:
Accepted:
Published:
Issue Date:
DOI: https://doi.org/10.1007/s10530-024-03363-w