Abstract
Three Geoid Slope Validation Surveys were planned by the National Geodetic Survey for validating geoid improvement gained by incorporating airborne gravity data collected by the “Gravity for the Redefinition of the American Vertical Datum” (GRAV-D) project in flat, medium and rough topographic areas, respectively. The first survey GSVS11 over a flat topographic area in Texas confirmed that a 1-cm differential accuracy geoid over baseline lengths between 0.4 and 320 km is achievable with GRAV-D data included (Smith et al. in J Geod 87:885–907, 2013). The second survey, Geoid Slope Validation Survey 2014 (GSVS14) took place in Iowa in an area with moderate topography but significant gravity variation. Two sets of geoidal heights were computed from GPS/leveling data and observed astrogeodetic deflections of the vertical at 204 GSVS14 official marks. They agree with each other at a \({\pm }1.2\,\, \hbox {cm}\) level, which attests to the high quality of the GSVS14 data. In total, four geoid models were computed. Three models combined the GOCO03/5S satellite gravity model with terrestrial and GRAV-D gravity with different strategies. The fourth model, called xGEOID15A, had no airborne gravity data and served as the benchmark to quantify the contribution of GRAV-D to the geoid improvement. The comparisons show that each model agrees with the GPS/leveling geoid height by 1.5 cm in mark-by-mark comparisons. In differential comparisons, all geoid models have a predicted accuracy of 1–2 cm at baseline lengths from 1.6 to 247 km. The contribution of GRAV-D is not apparent due to a 9-cm slope in the western 50-km section of the traverse for all gravimetric geoid models, and it was determined that the slopes have been caused by a 5 mGal bias in the terrestrial gravity data. If that western 50-km section of the testing line is excluded in the comparisons, then the improvement with GRAV-D is clearly evident. In that case, 1-cm differential accuracy on baselines of any length is achieved with the GRAV-D-enhanced geoid models and exhibits a clear improvement over the geoid models without GRAV-D data. GSVS14 confirmed that the geoid differential accuracies are in the 1–2 cm range at various baseline lengths. The accuracy increases to 1 cm with GRAV-D gravity when the west 50 km line is not included. The data collected by the surveys have high accuracy and have the potential to be used for validation of other geodetic techniques, e.g., the chronometric leveling. To reach the 1-cm height differences of the GSVS data, a clock with frequency accuracy of \(10^{-18}\) is required. Using the GSVS data, the accuracy of ellipsoidal height differences can also be estimated.












Similar content being viewed by others
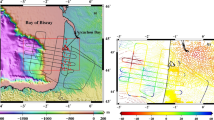
References
Bossler J (1984) FGCC standards and specifications for geodetic control networks. Library of Congress, Washington
Bürki B, Muller A, Kahle HG (2004) DIADEM: the new digital astronomical deflection measuring system for high-precision measurements of deflections of the vertical at ETH Ziirich. In: Proceedings IAG GGSM symposium, Porto, Portugal
Flury J (2016) Relativistic geodesy. In: Fritz R (ed) 8th symposium on frequency standards and metrology 2015. Journal of Physics: conference series 723:011001
Forsberg R (1984) A study of terrain reductions, density anomalies and geophysical inversion methods in gravity field modelling. Reports of the Department of Geodetic Science and Surveying, #355. The Ohio State University, Columbus
Guillaume S (2015) Determination of a precise gravity field for the CLIC feasibility studies. Ph.D. Thesis, Eidgenössische Technische Hochschule ETH Zürich, Nr. 22590. doi:10.3929/ethz-a-010549038
Heiskanen WA, Moritz H (1967) Physical geodesy. Freeman, San Francisco
Higgins MB, Pearse MB, Kearsley AHW (1996) Using digital elevation models in the computation of geoid heights. Geomat Res Aust 65:59–74
Hirt C (2004) Entwicklung und Erprobung eines digitalen Zenitkamerasystems für die hochpräzise Lotabweichungsbestimmung. Ph.D. thesis, Universität Hannover
Hirt C, Flury J (2008) Astronomical-topographic levelling using high-precision astrogeodetic vertical deflections and digital terrain model data. J Geod 82:231–248
Hoffmann-Wellenhof B, Moritz H (2006) Physical geodesy. Springer, Wien
Jekeli C (1999) An analysis of vertical deflections derived from high-degree spherical harmonic models. J Geoid 73:10–22
Jiang T, Wang YM (2016) On the spectral combination of satellite gravity model, terrestrial and airborne gravity data for local gravimetric geoid computation. J Geod 90:1405–1418
Li X, Crowley JW, Holmes SA, Wang YM (2016) The contribution of the GRAV-D airborne gravity to geoid determination in the Great Lakes region. Geophys Res Lett 43:4358–4365
Moritz H (1980) Advanced physical geodesy. Herbert Wichmann, Karlsruhe
Mayer-Gürr T et al (2012) The new combined satellite only model GOCO03s. SSHS2012, Venice
Mayer-Gürr T et al (2015) The combined satellite gravity field model GOCO05s. Presentation at EGU 2015, Vienna, April
Pavlis NK, Holmes SA, Kenyon SC, Factor JK (2012) The development and evaluation of the Earth Gravitational Model 2008 (EGM2008). J Geophys Res 117:B04406. doi:10.1029/2011JB008916
Smith DA, Holmes SA, Li XP, Guillaume S, Wang YM, Bürki B, Roman DR, Damiani TM (2013) Confirming regional 1 cm differential geoid accuracy from airborne gravimetry: the Geoid Slope Validation Survey of 2011. J Geod 87:885–907
Somieski AE (2008) Astrogeodetic geoid and isostatic considerations in the north Aegean sea, Greece. Ph.D. Thesis, Eidgenössische Technische Hochschule ETH Zürich, Nr. 17790
Wang YM, Li X, Holmes S, Roman DR, Smith DA (2013) Investigation of the use of deflections of vertical measured by DIADEM camera in the GSVS11 Survey, EGU General Assembly, held 7–12 April, 2013 in Vienna, Austria, id. EGU2013-12779
Wang YM, Weston ND, Mader G (2014) Verification of the accuracy of OPUS-projects ellipsoidal heights using GSVS11 leveling data and deflections of the vertical observed by the DIADEM Camera, April 17–May 02 EGU2014 Vienna, Austria
Wang YM, Martin D, Mader G (2016) Search results for: NGS finds real time solution in Iowa—Iowa RTN contributes to Geoid Slope Validation Survey of 2014, xyHt January 2016 Issue, p 24–27
Zilkoski DB, Richards JH, Young GM (1988) Results of the general adjustment of the North American Vertical Datum of 1988. Surv Land Inf Syst 52(3):133–149
Acknowledgements
The following NGS staffs participated in the survey and are sincerely acknowledged: Roy Anderson, James Richardson, Kendall Fancher, Don Breidenbach, T Hanson, Brian Shaw, Robert Hayes, Doug Adams, Courtney Lindo, Jim Tomlin, Simon Monroe, Eric Duvall, Tim Wilkins, Dan Callahan, Jim Harrington, Kevin Jordan, David Grosh, Phillip Marshall, Clyde Dean, Justin Dahlberg, Chris Villareal, Mark Schenewerk, Carly Weil, Simon Holmes, and Rick Foote. Discussions with Dr. Dru Smith and Mr. Jarir Saleh are important to improve the quality of the paper. The authors thank Dr. Kevin Ahgren for editing the paper.
Author information
Authors and Affiliations
Corresponding author
Appendices
Appendix 1: Errors of the DoV geoid profile
The errors in the DoV contain both systematic and random components. They affect the geoid accuracy differently. The systematic error has a much more profound impact on the geoid accuracy because it accumulates with respect to the length of the line linearly. In order to have a \(\pm 10\,\, \hbox {mm}\) geoid accuracy over a 325 km line, the systematic error in DoV has to be smaller than \(0.03\,\, \hbox {mm/km} = 0.0063^{\prime \prime }\). This is in the range of the systematic errors in a star catalog, anomalous refraction and others (Smith et al. 2013, Table 5). The error sources are difficult to locate and correct. An effective way to reduce the systematic error is to use a satellite gravity model combined with a high degree and order spherical harmonic coefficient model. Because the systematic error is (at least) very long wavelength, the satellite gravity models which are accurate in this frequency band can be used to control this type of error. The use of a high degree and order spherical harmonic coefficient model reduces the aliasing of high frequencies into lower frequencies of the gravity field.
Assuming the systematic error is removed, the remaining error \(\varepsilon (x)\) in DoV is only the random error which has the property
where \(E[\cdot ]\) is the expectation operator (Moritz 1980, p.76), \(\sigma _0^2 \) is the variance of random error in DoV data, and \(\delta (x,x^{\prime })\) is the delta function.
Ignoring the last term in Eq. (6) and assuming the geoid-quasigeoid separation term in (5) is free of error, the geoid error \(\theta (K)\) at the mark K can be expressed as
where d(K) is the distance from the starting point to the mark K.
The geoid error variance at mark K is
The root mean square of geoid error variance is then
Equation (15) shows the geoid error is linearly proportional to the square root of the length of the line. It is in the same form as the empirical error formula for spirit leveling (Zilkoski et al. 1988). If we take the random errors in the GSVS14 DoV as 0.05 arc second, Eq. (15) gives
where d is in km. This error is about one-third of the leveling of the first-order class II which has a formal accuracy of \(\pm 0.7\sqrt{d}\,\,\hbox {mm}\). The error computed from (16) increases gradually from few submillimeter to 17.0 mm for a 5000 km line crossing the US continent from west to east, if the systematic error is properly corrected. For the GSVS14 traverse (length of 320 km), the maximum error would be \(\pm 4.3\,\, \hbox {mm}\). This agrees quite well with the empirical results in Table 9 (Hirt and Flury 2008), taking into account the fact that CODIAC is nearly 50% more accurate than the camera used in their study.
Appendix 2: The Compact Digital Astrometric Camera CODIAC
The Compact Digital Astrometric Camera CODIAC (Fig. 13) is a new zenith camera system entirely designed, developed and manufactured at the Institute of Geodesy and Photogrammetry of ETH Zurich (Guillaume 2015). The principal objective behind the development of the new system was to replace the DIADEM with a system of reduced size and cost, based on commercial modern components, that provides the same level of accuracy as the DIADEM (Somieski 2008). In addition, it is designed with almost industrial standards in order to facilitate the use by non-astrogeodetic experts, to increase the performance in terms of productivity.
The two main components of the hardware consist of the astrometric (optical) part and the tilting part. The astrometric part is formed by a Riccardi-Honders Astrograph RH Veloce 200, manufactured by Officina Stellare, Italy. The unique optics have a focal length of 600 mm and an aperture of 216 mm, providing a focal ratio of f/3. In addition, the image acquisition is done by a CCD camera of FLI MicroLine KAF 8300 camera with an array of 3326 \(\times \) 2504 pixels of 5.4 \({\upmu }\)m providing an angular resolution of 1.86 arc second/pixel and a field of view of approximately \(1.2^{\circ } \times 1.6^{\circ }\). The global mechanical shutter is remotely triggered with a TTL signal generated by a ublox GNSS receiver.
The tiltmeter part is formed by two pairs of precise tiltmeters mounted orthogonally. The tilting part describing the relation between the optical rotation axis and the local plumb line consists of two pairs of tiltmeters. The first pair is of type Zerotronic manufactured by Wyler AG Winterthur, Switzerland. The second sensor pair consists of two high-resolution tiltmeters (HRTM), manufactured by Erich Lippmann, Schaufling, Germany. During the acquisition, the data are continuously recorded at a rate of 10 Hz.
The mechanical automation is done by 4 motors which control the extension of the electromechanical legs for the initial setup, the precise automatic levelling and the rotation of the superstructure into two faces.
The data acquisition on a station starts with an automatic levelling of the system at a level better than 5 arc second. Then, after checking the connections to the sensors and focusing, the data collection begins. The superstructure is rotated by 180\(^\circ \) around its vertical axis in order to eliminate most of the radial symmetric errors. In contrast to the DIADEM system (Bürki 2004), this setup is repeated 4 times. Each time the complete system is rotated by \(90^{\circ }\). This strategy attempts to eliminate residual systematic effects due to the mechanical angular variations which can appear when the superstructure is rotated. At the end of a station observation, approximately 150 sky-images are stored on the computer. Approximately 8000 stars are identified for each station (Fig. 14) and processed with the corresponding filtered tiltmeter.
The computation of the DoV is performed in the software AURIGA (Hirt 2004) whereas the tilt values are previously filtered, predicted, rectified with the calibration parameters (determined every day with a celestial calibration procedure) and combined in a least-squares collocation strategy. Prior to the final combination, the values from the Wyler and the Lippmann sensors can be compared. This comparison provides an independent check on tilt corrections (Fig. 15).
Rights and permissions
About this article
Cite this article
Wang, Y.M., Becker, C., Mader, G. et al. The Geoid Slope Validation Survey 2014 and GRAV-D airborne gravity enhanced geoid comparison results in Iowa. J Geod 91, 1261–1276 (2017). https://doi.org/10.1007/s00190-017-1022-1
Received:
Accepted:
Published:
Issue Date:
DOI: https://doi.org/10.1007/s00190-017-1022-1