Abstract
ATTR amyloidosis results from the conversion of transthyretin into amyloid fibrils that deposit in tissues causing organ failure and death. This conversion is facilitated by mutations in ATTRv amyloidosis, or aging in ATTRwt amyloidosis. ATTRv amyloidosis exhibits extreme phenotypic variability, whereas ATTRwt amyloidosis presentation is consistent and predictable. Previously, we found unique structural variabilities in cardiac amyloid fibrils from polyneuropathic ATTRv-I84S patients. In contrast, cardiac fibrils from five genotypically different patients with cardiomyopathy or mixed phenotypes are structurally homogeneous. To understand fibril structure’s impact on phenotype, it is necessary to study the fibrils from multiple patients sharing genotype and phenotype. Here we show the cryo-electron microscopy structures of fibrils extracted from four cardiomyopathic ATTRwt amyloidosis patients. Our study confirms that they share identical conformations with minimal structural variability, consistent with their homogenous clinical presentation. Our study contributes to the understanding of ATTR amyloidosis biopathology and calls for further studies.
Similar content being viewed by others
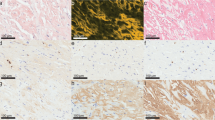
Introduction
Transthyretin amyloidosis (or ATTR amyloidosis) is a debilitating and progressive disorder caused by the abnormal build-up of transthyretin amyloid (ATTR) that leads to organ failure and death1. Transthyretin is primarily produced by the liver and secreted into the systemic circulation as a tetramer2. Aging or transthyretin mutations may trigger the dissociation of these tetramers into monomers, and the consequent formation of extracellular amyloid deposition3. In wild-type ATTR (ATTRwt) amyloidosis, these amyloids are composed of wild-type transthyretin and the clinical manifestation is primarily cardiomyopathy with late onset4,5. In heterozygous variant ATTR (ATTRv) amyloidosis, the deposits contain a mixture of both variant and wild-type transthyretin6,7, and the clinical manifestation is extremely variable, presenting a wide spectrum of symptoms such as cardiomyopathy and/or neuropathy, with ocular, gut, and/or kidney involvement, and unpredictable onsets3,4,8,9. Homozygous cases of ATTRv amyloidosis often present similar symptomatology as their heterozygous counterparts but with a more rapid disease progression and earlier onsets10. The molecular basis of the clinical discrepancies between ATTRwt and ATTRv amyloidosis patients remains elusive.
In amyloidoses of the central nervous system such as tauopathies and synucleinopathies, cryo-electron microscopy (cryo-EM) has revealed an association between the conformation of amyloid fibrils and the disease11,12,13,14. There are 68 ex-vivo structures of human brain amyloid fibrils determined to date (this number will likely be higher by the time of publication of this paper)12,15. Most of them feature the wild-type form of the amyloid precursor protein, with three exceptions12,15. One exception corresponds to fibrils extracted from the brain of an individual with juvenile-onset synucleinopathy, which contained both wild-type and a mutated form of α-synuclein16. The fibril structure in this case differs from those found in wild-type synucleinopathies14,16. A second one corresponds to fibrils extracted from two sibling patients that carry the deletion mutation ∆K281 in MAPT, leading to Pick’s disease17. The fibril structure in this case is identical to that of wild-type tau in Pick’s disease18. Worth noting, the tau isoform that is found in these fibrils does not include the R2 repeat, and therefore, the mutated residue11. The last exception corresponds to the prion fibril structure isolated from the brain of two Gerstmann–Sträussler–Scheinker disease (GSS) patients carrying the F198S mutation19. There is, however, no structures determined of wild-type prion fibrils from humans, and thus, their structures cannot be compared. Overall, it is safe to say that all structural studies on wild-type brain fibrils evidence the connection between individual diseases and their specific fibril structures. Inspired by those studies, we hypothesize that the more predictable and consistent phenotypic landscape in ATTRwt amyloidosis is associated with a structural homogeneity of ATTRwt fibrils.
Ten cryo-EM structures of ATTR fibrils have been reported to date7,20,21. Cardiac fibrils from five patients with ATTRv-V30M, ATTRv-T60A, ATTRv-V20I, ATTRv-G47E, ATTRv-V122I, and ATTRwt genetic backgrounds are structurally homogeneous, made of single protofilaments that share the same common core encompassing two fragments7,20,22,23,24. In all these structures, the C-terminal fragment folds into a channel made of polar residues that runs throughout the fibril. The remarkable similarity in fibril structures across different ATTR mutations may suggest additional unknown factors influencing the folding events during fibril formation. In contrast, in our recent studies we showed that cardiac fibrils from three polyneuropathic ATTRv-I84S patients and a polyneuropathic ATTRv-V122∆ patient display structural diversity, or polymorphism21,25. We observed that each of the three heterozygous ATTRv-I84S patients presents two polymorphs that can co-exist within the same fibril. One polymorph resembles the fold reported in cardiac ATTRwt or ATTRv structures, while the second polymorph shows local variation in the residues that close the polar channel (herein referred to as “the gate”)21. We found that these polymorphs with local structural variations are patient-specific. In turn, ATTRv-V122∆ fibrils are made of single and multiple protofilaments, each presenting the same conformation as observed in ATTRwt patients25. An additional study showed that fibrils extracted from the vitreous humor of an ATTRv-V30M patient are also highly polymorphic, with single, double, and triple filaments. The gate of the polar channel in the double filament ATTRv-V30M fibril structure from the eye adopts a distinct conformation, different from the one observed in the cardiac ATTRv-V30M structure7,26. These studies hint towards a possible structural heterogeneity in ATTRv fibrils that depends on phenotype (cardiomyopathy vs neuropathy), the location of fibril deposition, and/or the source of transthyretin since the source of transthyretin in the eye is the retina epithelium instead of the liver. Except for the ATTRv-I84S and ATTRv-T60A structures obtained from multiple patients, the other ATTR fibril structures were obtained from single patients, including one patient with ATTRwt amyloidosis7,20,21,22,24,25. The patient-specific polymorphism observed in ATTRv-I84S patients, which was not observed in different ATTRv-T60A patients, emphasizes the need to study the fibrils from multiple patients with the same phenotype and genotype.
In this study, we use cryo-EM to determine and analyze the structures of ex-vivo ATTRwt amyloid fibrils extracted from the hearts of four patients. Our findings confirm the highly structural uniformity in the ordered core of ATTRwt fibrils, sharing the same fold as the previously published ATTRwt fibril structure20. They also reveal a fully structured polar channel that appears perturbed in ATTRv-I84S fibrils from the heart and in V30M fibrils from the eye7,20,21,26. The structural homogeneity in ATTRwt fibrils may explain the consistent and predictable clinical presentation in patients, thereby suggesting a link between fibril structure and disease presentation in the case of ATTRwt amyloidosis, as established from previous observations of brain amyloid fibrils.
Results
Extraction and structure determination of ATTRwt fibrils
We successfully extracted the fibrils from the hearts of four patients with ATTRwt amyloidosis (patients ATTRwt-p1, -2, -3, and -4; details in Supplementary Table 1) using water-based extraction protocol7,21. We confirmed the protein identity and genotype using mass spectrometry (Supplementary Fig. 1, Supplementary Table 2). We observed various non-tryptic proteolytic sites in disordered regions as well as the amyloid core (Supplementary Fig 2). We also typed the samples using an antibody that recognizes C-terminal fragments of transthyretin and confirmed that the four ex-vivo extracts contained type A ATTR fibrils, which are made of both full-length and fragments of transthyretin (Supplementary Fig. 3a)27. For comparison, we used type B ATTR fibrils made of full-length transthyretin and no proteolytic fragments are observed. After extraction and validation, and before data collection, we screened ATTR fibrils for their purity and distribution by negative stain transmission electron microscopy (TEM) (Supplementary Fig. 3b) and cryo-EM. We found fibrils that appeared to be morphologically uniform in all four cases (Supplementary Fig. 4a). Fibrils were manually picked for helical reconstruction as described in the method section. As observed in our previous study on ATTRv-I84S fibrils, two-dimensional (2D) class averages discerned two fibril polymorphs: straight and curvy21. The straight species were unsuitable for structure determination because of their lack of twist. The curvy fibrils with a twisted structure were the most prevalent species (Supplementary Fig. 4b). We stitched together the representative 2D class averages of curvy fibrils and estimated their crossover distances (Supplementary Fig. 4c and Fig. 1). The three-dimensional (3D) class averaging of ATTRwt curvy fibrils resulted in a single class for all four patients with a visually similar structural arrangement of the amyloid core (Supplementary Fig. 4d). The overall resolution of the ATTRwt fibril structures from patients p1, p2, p3, and p4 are 3.31 Å, 3.28 Å, 3.32 Å and 3.43 Å, respectively (Supplementary Table 3, Supplementary Fig. 5 and Supplementary Fig. 6).
For each patient (a) ATTRwt-p1, (b) ATTRwt-p2, (c) ATTRwt-p3 and (d) ATTRwt-p4) figure shows the side view of the reconstructed fibril model with their crossover length (left), the closeup side view of the map with its helical rise (top right), and the top view of a single fibril layer with its model consisting N-terminal fragment (Pro 11/Leu 12 to Arg 34/Lys 35) and C-terminal fragment (Gly 57/Leu 58 to Thr 123/Asn 124) (bottom right.) The color coding is consistent in the cross-sectional model and in the side view of the 3D map. The dotted lines represent the missing residues (from 35/36 to 56/57) within the disordered region of ATTRwt structures.
Structural commonalities between ATTRwt fibrils
All four ATTRwt fibril structures share similar topology, with one protofilament consisting of two fragments: an N-terminal fragment from Pro 11/Leu 12 to Arg 34/Lys 35 and a C-terminal fragment from Leu 58 to Thr 123 (Fig. 1). The density corresponding to residues Ala 36 to His 56 was missing, suggesting lack of order or their absence. Both ATTRwt-p1 and p3 fibril structures have extra density for His31 rotamers, as previously seen in ATTRwt fibrils20, whereas ATTRwt-p2 and p4 fibril structures do not. In contrast to ATTRv-I84S fibrils21, the conformation of all ATTRwt fibril structures includes a polar channel that resembles a pentagon, starting at Leu 58 and closing at Ile 84. This channel is present in five other ATTR fibril structures from patients with cardiomyopathy or mixed phenotypes7,20,22.
The overall secondary structures of all ATTRwt fibrils including the fibril structure described in the study conducted by Steinebrei et al.20 (PDB 8ADE) show similar composition but are different from the native transthyretin (PDB 4TLT) (Fig. 2a). Each fibril layer consists of 14 β -strands (15 β -strands found in three of the four structures (Fig. 2b). The N-terminal fragment consists of three β -strands, with the third β -strand interdigitating with a C-terminal fragment forming what is known as a steric zipper28. The C-terminal fragment contains multiple β -strands that run along the fragment, with six of them involved in the formation of the polar channel. It is worth noting that only β -strands A, B, F, and H conserve their native secondary structure when converted into fibrils (Fig. 2a). This is of interest because β -strands F and H are the two amyloid-driving segments of transthyretin29, and β -strand B appears to be involved in protein oligomerization30,31.
a Primary sequence of wild-type transthyretin (top) with secondary structures of native-folded transthyretin (PDB 4TLT) and fibrils from four ATTRwt amyloidosis patients determined in this study (PDB codes 8E7D, 8G9R, 8GBR, and 8E7H) and previous study (PDB 8ADE). b Schematic view of the secondary structure on the fibril models of four ATTRwt fibrils.
The estimated solvation energies of four ATTRwt fibril structures are comparable to those of previously reported ATTRv fibrils32, averaging at −0.68 ± 0.08 kcal/mol per residue and −62.0 ± 6.5 kcal/mol per chain (Fig. 3a, Supplementary Fig. 7a and Supplementary Table 4). In comparison to the non-ATTR amyloid structures reported in the Amyloid Atlas, and similar to the ATTRv-I84S fibril structures we have recently reported, ATTRwt fibrils are estimated to be more stable, both by residue and chain15,21,33. The solvation energy estimates per residue (Fig. 3a and Supplementary Fig. 7a) and residue composition (Fig. 3b and Supplementary Fig. 7b) of ATTRwt fibrils revealed three major hydrophobic pockets contributing to the fibril stability. These pockets encompass the inner interface formed by the hairpin between residues Leu 12 and Val 32 in the N-terminal fragment, the aromatic pocket formed around residues Trp 79 and Phe 95 in the C-terminal fragment, and the triquetra that connects both C- and N- terminal fragments in the center of the structure core (Fig. 3b and Supplementary Fig. 7b). The structure is also stabilized by multiple backbone and side chain interactions, including hydrogen bonding and π-π stacking, as observed in ATTRv fibrils21.
a Representation of solvation energies per residue estimated from ATTRwt-p1 fibril structures determined in this study. Residues are colored from favorable (red, -2.5 kcal/mol) to unfavorable stabilization energy (blue, 2.5 kcal/mol). Scale, 10 Å. b Schematic view of ATTRwt-p1 fibril structures with residue composition. Residues are color-coded by amino acid category, as labeled. Scale, 10 Å.
Structural consistency of ATTRwt fibrils
We performed structural alignments of all published ATTR structures (Fig. 4a, b) and assessed backbone displacement (only the Ca atoms) through root mean square deviation (RMSD) calculations using GESAMT (Fig. 4c, d)34. Residues Leu 58 to Glu 66 within the gate region of the polar channel were excluded from analysis due to their contribution to ATTR fibril polymorphism, as previously discussed21. The structural alignment confirmed a consistent fold among all ATTRwt fibrils (Fig. 4a). Although the overall alignment of all structures suggests greater structural variability in variants to wild type, these differences are statistically non-significant (Fig. 4c). When analyzing the RMSD values by residue, ATTRwt-p1, -p3 and -p4 structures exhibit minimal variation with RMSD values through the protein sequence ranging from 0.338 to 0.680 Å. However, the ATTRwt-p2 structure deviates in the N-terminal region and the C-terminal spanning residues Trp 79 to Ser 100, with an overall RMSD of 1.26 Å (Fig. 4a, c and Supplementary Table 5). The ATTRv structures display greater variability in their backbone structures than ATTRwt structures, spanning from 0.478 to 1.944 (Supplement Table 6), with the highest values for ATTRv-I84S morphologies and the ATTRv-30M fibril structure from the eye (Supplementary Table 6 and Fig. 4b–d).
a Structure backbone alignment of ATTRwt fibrils with top view (top) and side view (bottom). ATTRwt-p1, in green, is used as a reference, and the other ATTRwt(s) are in light gray (three from this study and a published ATTRwt PDB 8ADE). b Structure backbone alignment of ATTRwt-p1 fibrils, in green; and other published ATTRv structures, in light pink (PDB codes 8E7E, 8E7J, 8E7I, 8TDN, 8TDO, 7OB4, 8PKG, 8PKE, 6SDZ, 8PKF). c Overall root mean square deviation (RMSD, only comparing Ca in Å) analysis of the structures included in (a) (ATTRwt—green circles) and (b) (ATTRv only—light pink squares) using GESAMT34. Lines indicate mean values. n is 5 for ATTRwt and 10 for ATTRv. ns- not significant. dDot plot of the RMSD analysis (only comparing Ca in Å) per residue from the same groups as in (c). Each dot represents the average RMSD compared to a consensus sequence, calculated by GESAMT for each group.
Discussion
In this study, we used cryo-EM to analyze the structures of ex-vivo ATTRwt amyloid fibrils extracted from the hearts of four patients. Our results confirm a highly uniform structure in ATTRwt fibrils, with a consistently ordered amyloid core and symmetry. Despite minor local variations, such as the extra density corresponding to His 31 rotamer in ATTRwt-p1 and -p2 fibrils, or higher RMSD values of ATTRwt-p2 in comparison to other ATTRwt structures, the overall secondary structure remains identical across all the ATTRwt fibril structures from various patients and demographics. This indicates a predictable fibril structure in ATTRwt amyloidosis. ATTRwt fibrils also show a range of crossover distances from 660 to 708 Å. Such differences suggest that the amyloid fibrils may accommodate certain level of flexibility without altering the core structure homogeneity, as evidenced by the similarity of secondary structure composition across ATTRwt fibrils.
Our findings add to the question about the potential association between fibril conformation and disease presentation in ATTR amyloidosis. As observed in the ATTRwt fibrils presented in this manuscript, ATTRv fibrils collected from the hearts of multiple patients reveal a common fibril conformation. Notably, the cardiac fibrils extracted from ATTRv-I84S and ATTRv-V122∆ fibrils display patient-specific local structural variations21,25. This raises the question of what differentiates these structurally homogeneous ATTRwt and ATTRv (V30M, V20I, G47E, V122I) fibrils from the heterogenous ATTRv-I84S and ATTRv-V122∆ fibrils. There are several potential explanations. One possibility is that the structural variability is mutation-driven. For instance, the mutation from Ile 84 to Ser 84 can disrupt the hydrophobic interaction with Leu 58 leading to local variations at the gate of the polar channel21. Another possibility is that the structural variability is associated with the phenotype, or vice versa. ATTRv-I84S and ATTRv-V122∆ amyloidoses present predominantly as polyneuropathy, whereas the other genotypes studied so far are associated with cardiomyopathy or a mixed phenotype. If the latter is correct, we anticipate that polyneuropathic genotypes will be associated with structural polymorphism whereas other cardiomyopathic and mixed ATTR genotypes will present a homogeneous structure (Supplementary Table 7). The structural homogeneity that we observe in ATTRwt fibrils extracted from four patients is consistent with both explanations. More structural and biological analyses are warranted to get a better understanding of the potential association between phenotype and fibril structure in ATTR amyloidosis.
Stemming from the results from previous studies on the structural dynamics of soluble transthyretin35,36,37,38, our cryo-EM observations, our current mass spectrometry analysis, and our previous studies on isolated transthyretin peptides29,39, we propose a potential aggregation pathway of transthyretin (Fig. 5). Our previous x-ray crystallography study shows that the β-strand B forms out-of-register amyloid fibrils, historically associated with oligomerization39,40,41,42,43. This strand and a portion of strand A are part of the N-terminal fragment of the mature ATTR fibril. We speculate that the N-terminal fragment first oligomerizes into a hairpin stabilized by a steric zipper formed in the interior of this hairpin (Fig. 5h). For this to happen, we propose that the segment from residue 36 to 62, approximately, encompassing strand C and D, would initially flip open to expose the N-terminal fragment from residue 11 to 35 (Fig. 5b, c). This proposal is consistent with the previous observation indicating that significant displacements of strands C and D, lead to the disruption of native contacts in the transthyretin structure35. This can be facilitated by proteolytic cleavage of residues within this segment, as observed in previous studies and our mass spectrometry analysis (Supplementary Fig. 2), or by mutations that destabilize the native structure29,30,36,37,38,39. In the native structure, the N-terminal fragment adopts a conformation close to a hairpin, so we speculate that the structural reorganization of this segment to convert into the fibril would likely be minimal. The rest of the monomer would then unfurl to open into two wings. The C-terminal fragment from Gly 67 to Thr 123 would reorganize forming the rest of the core (Fig. 5d–g) followed by the folding of the gate. Notably, several cleavage sites occur at the pivotal points of the fibril structure where Pro and/or Gly are present, suggesting a potential role during protein folding into the final structure of ATTR fibril (Supplementary Fig. 2). We speculate that transthyretin mutations are more likely to affect the folding of the fibrils in the last steps, leading to structural polymorphism. This structural polymorphism could potentially contribute to organ tropism, proteolysis susceptibility, seeding ability, and other features of pathological importance.
a Tetrameric transthyretin (PDB 4TLT). b Monomer unit after dissociating from tetrameric form. c Strands C and D flip away to expose strands A and B. d Strands A and B form hairpin structure while strand E and helix unwind to shape into the core of the polar channel. e Strands G and H rotate to approximately 135° to strand F, so strand F is now free to interact with the back of the polar channel through the formation of an aromatic pocket. f The loop region of strand H and the C-terminal part of strand G unfolds to form the triquetra connecting the N-terminal hairpin and the back of the polar channel. g Strand H rotates to break the β-sheet hydrogen bonds with strand G and create new interaction at the back of strand G. h Top view of a single layer of the mature ATTR fibril. Color codes represent different regions of the ATTR fibril and monomeric transthyretin. Red, N-terminal hairpin. Purple, unstructured region encompassing strands C and D, which connects the N-terminal to the C-terminal fragments of the ATTR fibril. Pink, the loop between strands D and E that form the gate of the polar channel. Green, strands E, F, G, and H that form the rest of the C-terminal fragment of the ATTR fibril.
While this study provides important insights into the structure of ATTRwt amyloid fibrils, there is still much to be learned about the structural basis of ATTR amyloidosis. Future research could explore the relationship between fibril structure and disease severity, as well as investigate the mechanisms underlying the early steps in fibril formation and propagation. Together, the understanding of the structural basis of ATTR amyloidosis will enable a more accurate identification of the disease and the identification of novel targets for therapeutics and diagnostics development.
Methods
Patients and tissue material
We obtained fresh frozen cardiac tissues from ATTRwt amyloidosis patients (n = 4). All samples were postmortem. The details of each tissue sample included in the study are listed in Supplementary Table 1. Specimens from the left ventricle of either explanted or autopsied hearts were obtained from the laboratory of the late Dr. Merrill D. Benson at the University of Indiana. The Office of the Human Research Protection Program granted expedited approval from the Internal Review Board review because all specimens were anonymized. All ethical regulations relevant to human research participants were followed.
Extraction of amyloid fibrils from human cardiac tissue
Ex-vivo preparations of amyloid fibrils were obtained from the fresh-frozen human tissue7,21. Briefly, ~200 mg of frozen cardiac tissue per patient was thawed at room temperature and cut into small pieces with a scalpel. The minced tissue was resuspended into 1 mL Tris-calcium buffer (20 mM Tris, 138 mM NaCl, 2 mM CaCl2, 0.1% NaN3, pH 8.0) and centrifuged for 5 min at 3100 × g and 4 °C. The pellet was washed in Tris-calcium buffer four additional times. After the washing, the pellet was resuspended in 1 mL of 5 mg mL−1 collagenase solution (collagenase stock prepared in Tris-calcium buffer) and incubated overnight at 37 °C, shaking at 400 rpm. The resuspension was centrifuged for 30 min at 3100 × g and 4 °C and the pellet was resuspended in 1 mL Tris–ethylenediaminetetraacetic acid (EDTA) buffer (20 mM Tris, 140 mM NaCl, 10 mM EDTA, 0.1% NaN3, pH 8.0). The suspension was centrifuged for 5 min at 3100 × g and 4 °C, and the washing step with Tris–EDTA was repeated nine additional times. All the supernatants were collected for further analysis, when needed. After the washing, the pellet was resuspended in 200 μL ice-cold water supplemented with 5 mM EDTA and centrifuged for 5 min at 3100 × g and 4 °C. This step released the amyloid fibrils from the pellet, which were collected in the supernatant. EDTA helped solubilize the fibrils. This extraction step was repeated three additional times. The material from the various patients was handled and analyzed separately.
Negative-stained transmission electron microscopy
The presence of amyloid fibrils in the elution was confirmed by transmission electron microscopy21. Briefly, a 3 μL sample was spotted onto a freshly glow-discharged carbon film 300 mesh copper grid (Electron Microscopy Sciences), incubated for 2 min, and gently blotted onto a filter paper to remove the solution. The grid was negatively stained with 5 µL of 2% uranyl acetate for 2 min and gently blotted to remove the solution. Another 5 μL uranyl acetate was applied onto the grid and immediately removed. An FEI Tecnai 12 electron microscope at an accelerating voltage of 120 kV was used to examine the specimens.
Western blotting of extracted ATTR fibrils
Western blotting was performed on the extracted fibrils to confirm fibril type. Briefly, 0.5 µg fibrils were dissolved in a Tricine SDS sample buffer, boiled for 2 minutes at 85 °C, and run on a Novex™ 16% Tris-tricine gel system using a Tricine SDS running buffer. ATTR fibril type was determined by transferring the gel contents to a 0.2 µm nitrocellulose membrane and probing with a primary antibody (1:1000) directed against the C-terminal region of the wild type TTR sequence (GenScript). Horseradish peroxidase-conjugated goat anti-rabbit IgG (Invitrogen, at 1:1000) was used as a secondary antibody. Promega Chemiluminescent Substrate (Promega) was used according to the manufacturer’s instructions to visualize ATTR content.
Mass Spectrometry (MS) sample preparation, data acquisition and analysis
For tryptic MS analysis, 0.5 µg of extracted ATTR fibrils were dissolved in a Tricine SDS sample buffer, boiled for 2 min at 85 °C, and run on a Novex™ 16% tris-Tricine gel system using a Tricine SDS running buffer. The gel was stained with Coomassie dye, destained and ATTR smear was cut from the gel. Sample was sent for MS analysis. Samples were digested overnight with trypsin (Pierce) following reduction and alkylation with DTT and iodoacetamide (Sigma–Aldrich). The samples then underwent solid-phase extraction cleanup with an Oasis HLB plate (Waters) and the resulting samples were injected onto an Q Exactive HF mass spectrometer coupled to an Ultimate 3000 RSLC-Nano liquid chromatography system. Samples were injected onto a 75 um i.d., 15-cm long EasySpray column (Thermo) and eluted with a gradient from 0-28% buffer B over 90 min. Buffer A contained 2% (v/v) ACN and 0.1% formic acid in water, and buffer B contained 80% (v/v) ACN, 10% (v/v) trifluoroethanol, and 0.1% formic acid in water. The mass spectrometer operated in positive ion mode with a source voltage of 2.5 kV and an ion transfer tube temperature of 300 °C. MS scans were acquired at 120,000 resolution in the Orbitrap and up to 20 MS/MS spectra were obtained in the ion trap for each full spectrum acquired using higher-energy collisional dissociation (HCD) for ions with charges 2-8. Dynamic exclusion was set for 20 s after an ion was selected for fragmentation.
Raw MS data files were analyzed using Proteome Discoverer v3.0 SP1 (Thermo), with peptide identification performed using a semitryptic search with Sequest HT against the human-reviewed protein database from UniProt. Fragment and precursor tolerances of 10 ppm and 0.02 Da were specified, and three missed cleavages were allowed. Carbamidomethylation of Cys was set as a fixed modification, with oxidation of Met set as a variable modification. The false-discovery rate (FDR) cutoff was 1% for all peptides.
Cryo-EM sample preparation, data collection, and processing
Freshly extracted fibril samples were applied to glow-discharged Quantifoil R 1.2/1.3, 300 mesh, Cu grids, blotted with filter paper to remove excess sample, and plunged frozen into liquid ethane using a Vitrobot Mark IV (FEI). Cryo-EM samples were screened on either the Talos Arctica or Glacios at the Cryo-Electron Microscopy Facility (CEMF) at The University of Texas Southwestern Medical Center (UTSW), and the final datasets were collected on a 300 kV Titan Krios microscope (FEI) equipped with K3 detector at the Stanford-SLAC Cryo-EM Center (S2C2) (Table 1). Pixel size, frame rate, dose rate, final dose, and number of micrographs per sample are detailed in Table 1. Automated data collection was performed by SerialEM software package44 and Thermo Scientific Smart EPU software. The raw movie frames were gain-corrected, aligned, motion-corrected and dose-weighted using RELION’s own implemented motion correction program45. Contrast transfer function (CTF) estimation was performed using CTFFIND 4.146. All steps of helical reconstruction, three-dimensional (3D) refinement, and post-process were carried out using RELION 3.147. All filaments were manually picked using EMAN2 e2helixboxer.py48. Particles were extracted using a box size of 1024 and 256 pixels with an inter-box distance of 10% of the box length. 2D classification of 1024-pixel particles was used to estimate the helical parameters. 2D classifications of 256-pixel particles were used to select suitable particles for further processing. Fibril helix is assumed to be left-handed as the resolution limits the interpretation of the handedness. We used an elongated Gaussian blob as an initial reference for 3D classifications of ATTRwt-p1, ATTRwt-p2 and ATTRwt-p4. For ATTRwt-p3, we used a RELION built-in script, relion_helix_inimodel2d, to generate an incomplete initial model and low-pass filtered the model to 50 Å. We performed 3D classifications with an average of ~30k to 40k particles per class to separate filament types. Particles potentially leading to the best reconstructed map were chosen for 3D auto-refinements. CTF refinements and Bayesian polishing were performed to obtain higher resolution. Final maps were post-processed using the recommended standard procedures in RELION 3.1. The final subset of selected particles was used for high-resolution gold-standard refinement49. The final overall resolution estimate was evaluated based on the FSC at 0.143 threshold between two independently refined half-maps (Supplementary Fig. 6)50.
Model building
The refined maps were post-processed in RELION before building their models51. Our previously published model of ATTRv-P24S (PDB code 8E7I) was used as the template to build the model of ATTRwt-p1, which in turn, was used as a template to build the other ATTRwt models. Residue modification, rigid body fit zone, and real space refine zone were performed to obtain the resulting models using COOT52. All the statistics are summarized in Table 1.
Stabilization energy calculation
The stabilization energy per residue was calculated by the sum of the products of the area buried for each atom and the corresponding atomic solvation parameters (Supplementary Fig. 7a)15,21,53. The overall energy was calculated by the sum of energies of all residues, and assorted colors were assigned to each residue, instead of each atom, in the solvation energy map.
Statistics and reproducibility
RMSD data in Cα position per protein structure and per residue were calculated by GESAMT34,54. Statistical analysis of fibril stability and RMSD in Cα atoms were performed with Prism 9 for Mac (GraphPad Software) using an unpaired t test. Number n is 5 for ATTRwt and 10 for ATTRv. ns- not significant. All samples were included in the analysis and all measurements are displayed in the graphs.
Figure Panels. Figure panels were created with ChimeraX 1.6, Pymol 2.3.5 and Adobe Illustrator 2020.
Data availability
Structural data have been deposited into the Worldwide Protein Data Bank (wwPDB) and the Electron Microscopy Data Bank (EMDB) with the following EMD accession codes: 26587 (ATTRwt-p1), 29874 (ATTRwt-p2), 29920 (ATTRwt-p3), 26691 (ATTRwt-p4), and PDB accession codes: 8E7D (ATTRwt-p1), 8G9R (ATTRwt-p2), 8GBR (ATTRwt-p3), 8E7H (ATTRwt-p4). The PDB accession code for the previously reported coordinates of ATTRT60A used for data processing is 8E7G. The mass spectrometry proteomics data have been deposited to MassIVE data repository, with accession number MSV000094066 ftp://MSV000094066@massive.ucsd.edu. All data generated or analyzed during this study that support the findings are available within this published article and its supplementary data files. The source data behind the graphs in Fig. 4c, d are shown in Supplementary Data 1. Cardiac specimens were obtained from the laboratory of the late Dr. Merrill D. Benson at Indiana University. These specimens are under a material transfer agreement with Indiana University and cannot be distributed freely.
References
Tschöpe, C. & Elsanhoury, A. Treatment of transthyretin amyloid cardiomyopathy: the current options, the future, and the challenges. J. Clin. Med. 11, 2148 (2022).
Buxbaum, J. N. & Reixach, N. Transthyretin: the servant of many masters. Cell Mol. Life Sci. 66, 3095–3101 (2009).
Bezerra, F., Saraiva, M. J. & Almeida, M. R. Modulation of the mechanisms driving transthyretin amyloidosis. Front. Mol. Neurosci. 13, 592644 (2020).
González-López, E. et al. Wild-type transthyretin amyloidosis as a cause of heart failure with preserved ejection fraction. Eur. Heart J. 36, 2585–2594 (2015).
Narotsky, D. L., Castano, A., Weinsaft, J. W., Bokhari, S. & Maurer, M. S. Wild-type transthyretin cardiac amyloidosis: novel insights from advanced imaging. Can. J. Cardiol. 32, 1166.e1161–1166.e1110 (2016).
Ihse, E., Suhr, O. B., Hellman, U. & Westermark, P. Variation in amount of wild-type transthyretin in different fibril and tissue types in ATTR amyloidosis. J. Mol. Med. 89, 171–180 (2011).
Schmidt, M. et al. Cryo-EM structure of a transthyretin-derived amyloid fibril from a patient with hereditary ATTR amyloidosis. Nat. Commun. 10, 5008 (2019).
Dispenzieri, A. et al. Clinical and genetic profile of patients enrolled in the Transthyretin Amyloidosis Outcomes Survey (THAOS): 14-year update. Orphanet. J. Rare Dis. 17, 236 (2022).
Brunjes, D. L., Castano, A., Clemons, A., Rubin, J. & Maurer, M. S. Transthyretin cardiac amyloidosis in older Americans. J. Card. Fail. 22, 996–1003 (2016).
Hood, C. J. et al. Update on disease-specific biomarkers in transthyretin cardiac amyloidosis. Curr. Heart Fail. Rep. 19, 356–363 (2022).
Falcon, B. et al. Tau filaments from multiple cases of sporadic and inherited Alzheimer’s disease adopt a common fold. Acta Neuropathol. 136, 699–708 (2018).
Shi, Y. et al. Structure-based classification of tauopathies. Nature 598, 359–363 (2021).
Yang, Y. et al. Cryo-EM structures of amyloid-β 42 filaments from human brains. Science 375, 167–172 (2022).
Yang, Y. et al. Structures of α-synuclein filaments from human brains with Lewy pathology. Nature 610, 791–795 (2022).
Sawaya, M. R., Hughes, M. P., Rodriguez, J. A., Riek, R. & Eisenberg, D. S. The expanding amyloid family: structure, stability, function, and pathogenesis. Cell 184, 4857–4873 (2021).
Yang, Y. et al. New SNCA mutation and structures of α-synuclein filaments from juvenile-onset synucleinopathy. Acta Neuropathol. 145, 561–572 (2023).
Schweighauser, M. et al. Mutation ∆K281 in MAPT causes Pick’s disease. Acta Neuropathol. 146, 211–226 (2023).
Falcon, B. et al. Structures of filaments from Pick’s disease reveal a novel tau protein fold. Nature 561, 137–140 (2018).
Hallinan, G. I. et al. Cryo-EM structures of prion protein filaments from Gerstmann–Sträussler–Scheinker disease. Acta Neuropathol. 144, 509–520 (2022).
Steinebrei, M. et al. Cryo-EM structure of an ATTRwt amyloid fibril from systemic non-hereditary transthyretin amyloidosis. Nat. Commun. 13, 6398 (2022).
Nguyen, B. A. et al. Structural polymorphism of amyloid fibrils in ATTR amyloidosis revealed by cryo-electron microscopy. Nat. Commun. 15, 581 (2024).
Steinebrei, M. et al. Common transthyretin-derived amyloid fibril structures in patients with hereditary ATTR amyloidosis. Nat. Commun. 14, 7623 (2023).
Nguyen, B. A. et al. ATTRv-V30M Type A amyloid fibrils from heart and nerves exhibit structural homogeneity. bioRxiv https://doi.org/10.1101/2024.05.14.594028 (2024).
Fernandez-Ramirez, M. D. C. et al. Multi-organ structural homogeneity of amyloid fibrils in ATTRv-T60A amyloidosis patients, revealed by Cryo-EM. bioRxiv https://doi.org/10.1101/2024.05.14.594218 (2024).
Ahmed, Y. et al. Amyloid fibril polymorphism in the heart of an ATTR amyloidosis patient with polyneuropathy attributed to the V122Δ variant. bioRxiv https://doi.org/10.1101/2024.05.09.593396 (2024).
Iakovleva, I. et al. Structural basis for transthyretin amyloid formation in vitreous body of the eye. Nat. Commun. 12, 7141 (2021).
Ihse, E. et al. Amyloid fibrils containing fragmented ATTR may be the standard fibril composition in ATTR amyloidosis. Amyloid 20, 142–150 (2013).
Nelson, R. et al. Structure of the cross-β spine of amyloid-like fibrils. Nature 435, 773–778 (2005).
Saelices, L. et al. Uncovering the mechanism of aggregation of human transthyretin. J. Biol. Chem. 290, 28932–28943 (2015).
Saelices, L. et al. Amyloid seeding of transthyretin by ex vivo cardiac fibrils and its inhibition. Proc. Natl Acad. Sci. USA 115, E6741–E6750 (2018).
Stevens, K. R. et al. In situ expansion of engineered human liver tissue in a mouse model of chronic liver disease. Sci. Transl. Med. 9, eaah5505 (2017).
Nguyen, B. A., et al. Structural polymorphism of amyloid fibrils in cardiac transthyretin amyloidosis revealed by cryo-electron microscopy. bioRxiv https://doi.org/10.1101/2022.06.21.496949 (2022).
Sawaya, M. R. Amyloid Atlas 2024. https://people.mbi.ucla.edu/sawaya/amyloidatlas/ (2024).
Krissinel, E. Enhanced fold recognition using efficient short fragment clustering. J. Mol. Biochem 1, 76–85 (2012).
Yee, A. W. et al. A molecular mechanism for transthyretin amyloidogenesis. Nat. Commun. 10, 925 (2019).
Marcoux, J. et al. A novel mechano‐enzymatic cleavage mechanism underlies transthyretin amyloidogenesis. EMBO Mol. Med. 7, 1337–1349 (2015).
Hurshman Babbes, A. R., Powers, E. T. & Kelly, J. W. Quantification of the thermodynamically linked quaternary and tertiary structural stabilities of transthyretin and its disease-associated variants: the relationship between stability and amyloidosis. Biochemistry 47, 6969–6984 (2008).
Ihse, E. et al. Proportion between wild-type and mutant protein in truncated compared to full-length ATTR: an analysis on transplanted transthyretin T60A amyloidosis patients. Biochem. Biophys. Res. Commun. 379, 846–850 (2009).
Saelices, L., Sievers, S. A., Sawaya, M. R. & Eisenberg, D. S. Crystal structures of amyloidogenic segments of human transthyretin. Protein Sci. 27, 1295–1303 (2018).
Sangwan, S. et al. Atomic structure of a toxic, oligomeric segment of SOD1 linked to amyotrophic lateral sclerosis (ALS). Proc. Natl Acad. Sci. USA 114, 8770–8775 (2017).
Soriaga, A. B., Sangwan, S., Macdonald, R., Sawaya, M. R. & Eisenberg, D. Crystal structures of IAPP amyloidogenic segments reveal a novel packing motif of out-of-register beta sheets. J. Phys. Chem. B 120, 5810–5816 (2016).
Liu, C. et al. Out-of-register β-sheets suggest a pathway to toxic amyloid aggregates. Proc. Natl Acad. Sci. USA 109, 20913–20918 (2012).
Lövestam, S. et al. Disease-specific tau filaments assemble via polymorphic intermediates. Nature 625, 119–125 (2024).
Mastronarde, D. N. Automated electron microscope tomography using robust prediction of specimen movements. J. Struct. Biol. 152, 36–51 (2005).
Zivanov, J., Nakane, T. & Scheres, S. H. W. A Bayesian approach to beam-induced motion correction in cryo-EM single-particle analysis. IUCrJ 6, 5–17 (2019).
Rohou, A. & Grigorieff, N. CTFFIND4: Fast and accurate defocus estimation from electron micrographs. J. Struct. Biol. 192, 216–221 (2015).
He, S. & Scheres, S. H. W. Helical reconstruction in RELION. J. Struct. Biol. 198, 163–176 (2017).
Bell, J. M., Chen, M., Durmaz, T., Fluty, A. C. & Ludtke, S. J. New software tools in EMAN2 inspired by EMDatabank map challenge. J. Struct. Biol. 204, 283–290 (2018).
Scheres, S. H. W. RELION: Implementation of a Bayesian approach to cryo-EM structure determination. J. Struct. Biol. 180, 519–530 (2012).
Chen, S. et al. High-resolution noise substitution to measure overfitting and validate resolution in 3D structure determination by single particle electron cryomicroscopy. Ultramicroscopy 135, 24–35 (2013).
Terwilliger, T. C., Sobolev, O. V., Afonine, P. V. & Adams, P. D. Automated map sharpening by maximization of detail and connectivity. Acta Crystallogr. D: Struct. Biol. 74, 545–559 (2018).
Emsley, P., Lohkamp, B., Scott, W. G. & Cowtan, K. Features and development of Coot. Acta Crystallogr. D: Biol. Crystallogr. 66, 486–501 (2010).
Eisenberg, D. & McLachlan, A. D. Solvation energy in protein folding and binding. Nature 319, 199–203 (1986).
Winn, M. D. et al. Overview of the CCP4 suite and current developments. Acta Crystallogr. D: Biol. Crystallogr. 67, 235–242 (2011).
Acknowledgements
In memory of the Late Dr. Merrill D. Benson, who contributed greatly to the understanding of amyloid diseases and helped affected families for decades. Special thanks to the patients and families who generously donated tissues and the University of Indiana as the source of material. We thank Dr. Michael Sawaya for his answers and invaluable advice. We thank SBWIP, UTSW for the constructive discussions and valuable comments. valuable We thank the UTSW Cryo-Electron Microscopy Facility, the UTSW Structural Biology Laboratory, the UTSW Electron Microscopy Core Facility, the national cryo-EM facilities Stanford-SLAC (project CA60) and PNCC (project 51267) for instrumentation, technical support, and/or data collection. We thank UTSW Proteomics core for technical assistance with the proteomics experiments. This study was supported by the following funding bodies: American Heart Association, Career Development Award 847236, L.S. National Institutes of Health, National Heart, Lung, and Blood Institute, New Innovator Award DP2-HL163810, L.S. Welch Foundation, Research Award I-2121-20220331, L.S. UTSW Endowment, Distinguished Researcher Award from President’s Research Council and start-up funds, L.S. Cryo-EM research was partially supported by the following grants: National Institutes of Health grant U24GM129547, Department of Energy Office of Science User Facility sponsored by the Office of Biological and Environmental Research. Department of Energy, Laboratory Directed Research and Development program at SLAC National Accelerator Laboratory, under contract DE-AC02-76SF00515 NIH Common Fund Transformative High Resolution Cryo-Electron Microscopy program (U24 GM129539). The Cryo-Electron Microscopy Facility and the Structural Biology Laboratory at UTSW are supported by a grant from the Cancer Prevention & Research Institute of Texas (RP170644). The Electron Microscopy Core Facility at UTSW is supported by the National Institutes of Health (NIH (1S10OD021685-01A1 and 1S10OD020103-01). Part of the computational resources were provided by the BioHPC supercomputing facility located in the Lyda Hill Department of Bioinformatics at UTSW. URL: https://portal.biohpc.swmed.edu.
Author information
Authors and Affiliations
Contributions
Conceptualization: B.N., L.S. Methodology: B.N., V.S., L.S. Investigation: B.N., V.S. S.A., P.S., M.P., Y.A., R.P., J.C., A.L., B.K., P.M.W., F.C., L.S. Visualization: B.N., V.S., L.S. Funding acquisition: L.S. Project administration L.S. Supervision: B.N., V.S., L.S. Writing—original draft: L.S. Writing—review & editing: B.N., V.S., S.A., Y.A., R.P., L.S.
Corresponding author
Ethics declarations
Competing interests
L.S. consults for Intellia Therapeutics Inc. and Attralus Inc., and Advisory Board member for Alexion Pharmaceuticals. The remaining authors declare no competing interests.
Peer review
Peer review information
Communications Biology thanks Jeffrey Savas, Sung-Tsang Hsieh and the other, anonymous, reviewer(s) for their contribution to the peer review of this work. Primary Handling Editor: Manuel Breuer. [A peer review file is available.]. This manuscript has been previously reviewed at another Nature Portfolio journal. This document only contains reviewer comments and rebuttal letters for versions considered at Communications Biology.
Additional information
Publisher’s note Springer Nature remains neutral with regard to jurisdictional claims in published maps and institutional affiliations.
Rights and permissions
Open Access This article is licensed under a Creative Commons Attribution-NonCommercial-NoDerivatives 4.0 International License, which permits any non-commercial use, sharing, distribution and reproduction in any medium or format, as long as you give appropriate credit to the original author(s) and the source, provide a link to the Creative Commons licence, and indicate if you modified the licensed material. You do not have permission under this licence to share adapted material derived from this article or parts of it. The images or other third party material in this article are included in the article’s Creative Commons licence, unless indicated otherwise in a credit line to the material. If material is not included in the article’s Creative Commons licence and your intended use is not permitted by statutory regulation or exceeds the permitted use, you will need to obtain permission directly from the copyright holder. To view a copy of this licence, visit http://creativecommons.org/licenses/by-nc-nd/4.0/.
About this article
Cite this article
Nguyen, B.A., Singh, V., Afrin, S. et al. Cryo-EM confirms a common fibril fold in the heart of four patients with ATTRwt amyloidosis. Commun Biol 7, 905 (2024). https://doi.org/10.1038/s42003-024-06588-6
Received:
Accepted:
Published:
DOI: https://doi.org/10.1038/s42003-024-06588-6
- Springer Nature Limited