Abstract
Nitrous oxide (N2O) is formed during wastewater nitrogen removal processes. It is a strong greenhouse gas, however, if properly captured it can also be used as a renewable energy source. In this study, a nosZ-deficient strain of Pseudomonas aeruginosa was constructed. During growth under denitrifying conditions, the nosZ-deficient strain was more highly transcribing other genes from the denitrification pathway (narG, nirS, and norB) than the wild-type strain. This strain could also convert 85% of NO2−-N to N2O when it was grown with acetate compared to <0.6% by the wild-type strain. When a bioreactor treating synthetic wastewater with high NO2−-N concentrations (700 mg/L) was inoculated with this strain, the N2O conversion efficiencies were >73% and N2O comprised 73~81% of the biogas being generated. The energy yield from wastewater in bioaugmented reactors also reached levels as high as 1260 kJ/m3. These results are significant and show that bioaugmentation of reactors during denitrification treatment processes with nosZ-deficient strains of Pseudomonas or other core denitrifying bacteria might be an effective way to enhance N2O recovery.
Similar content being viewed by others
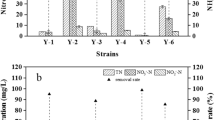
Introduction
Nitrous oxide (N2O) is a strong greenhouse gas with an atmospheric lifetime of ~120 years and a greenhouse effect that is 296 times stronger than CO21. A significant proportion of global N2O emissions (3.2–10%) can be attributed to nitrogen removal processes carried out during wastewater treatment2,3,4,5,6. Although N2O has many negative impacts if released into the environment, recent studies have shown that N2O can serve as a renewable energy source7,8. In fact, N2O has a positive enthalpy of formation, and can release 82 kJ/mol when decomposed (eqn. 1). N2O is also a more powerful oxidant in combustion reactions than O2. For example, stoichiometric combustion of 1 mol CH4 with N2O releases roughly 30% more energy than stoichiometric combustion of 1 mol CH4 with O29 (eqns 2–3).
Therefore, N2O is commonly used to supercharge the engines of high performance vehicles (i.e. “Nitrox”) and as an oxidant in hybrid rocket motors in the aerospace industry9.
Researchers have started to examine the possibility of stimulating N2O emissions from bioreactors undergoing wastewater nitrogen removal treatment processes4,9,10,11,12. Many nitrogen removal treatment approaches utilize bacteria present in wastewater that can reduce nitrate or nitrite to nitrogen gas under anaerobic conditions13. N2O is an intermediate in most denitrification pathways11 and many researchers have tried to adjust operational parameters in bioreactors to maximize N2O output12,14,15,16,17,18,19. Unfortunately, these attempts have resulted in low conversion rates of total nitrogen to N2O (usually <10%), or unstable reactors4,11,12. It does not help that the solubility of N2O in pure water is extremely high at room temperature (i.e. 1500 mg/L)20. Therefore, when wastewater with low total nitrogen content (i.e. municipal wastewater) is treated, the majority of N2O is dissolved in the aqueous phase and flows out with the effluent.
One promising strategy called Coupled Aerobic–anoxic Nitrous Decomposition Operation (CANDO) utilizes a three-step process to remove nitrogen from wastewater while generating N2O that is then used for catalytic decomposition to N2 and O2 or as an oxidant for CH4 combustion9. This approach involves the partial nitrification of NH4+ to NO2− which is then anaerobically reduced to N2O. Scherson, et al.9 found that if they supplemented reactors with pulses of electron donor (acetate) followed by pulses of electron acceptor (NO2−) during the denitrification stage of treatment, they could suppress the growth of complete denitrifiers (N2-producing) while slightly stimulating the growth of incomplete denitrifiers (N2O-producing) and polyhydroxybutyrate (PHB) accumulating denitrifiers that could couple the oxidation of PHB with nitrite reduction. This approach resulted in an N2O conversion efficiency of 60–65%, however, the majority of N2O was dissolved in the aqueous phase making energy recovery difficult.
Some denitrifying bacteria from such genera as Pseudoxanthomonas21, Stenotrophomonas22, Luteimonas22, Corynebacterium23 and Moraxella24, have a truncated denitrification pathway and their chromosomes lack the gene coding for nitrous oxide reductase (NosZ) which means that N2O is their terminal denitrification product. However, conditions maintained in wastewater treatment reactors are not optimal for growth of these species, and they are seldomly enriched during wastewater treatment25,26. It seems much more practical to focus efforts on organisms that are known to be significant members of the wastewater community during nitrogen removal treatment processes25,26.
Pseudomonas aeruginosa is one example of a denitrifying bacterium that is frequently enriched in wastewater treatment plants and bioreactors9,27,28. Although P. aeruginosa is a complete denitrifier (nitrogen metabolism pathways of P. aeruginosa are shown in Fig. 1), it is genetically tractable and can survive when the nosZ gene is deleted from its chromosome29. Therefore, a lab-scale moving-bed biofilm reactor (MBBR) system treating high strength nitrogen synthetic wastewater was bioaugmented with a nosZ-deficient strain of P. aeruginosa. Significant concentrations of N2O were obtained in biogas generated by this system and N2O conversion efficiencies were higher than previously reported studies.
Results
Enhanced N2O production by nosZ-deficient mutant strain of Pseudomonas aeruginosa in pure culture studies
Nitrous oxide is formed as an intermediate by P. aeruginosa during denitrification (Fig. 1). Nitrate is first reduced by nitrate reductase (NarGHI) to nitrite, which is then reduced to nitric oxide by nitrite reductase (NirS). Nitric oxide is reduced by nitric oxide reductase (NorBC) to nitrous oxide and finally nitrous oxide is converted to nitrogen gas by nitrous-oxide reductase (NosZ). In an attempt to halt the pathway at nitrous oxide formation, nosZ-deletion mutant strains were constructed.
The formation of nitrous oxide over time was measured in cultures of nosZ-deficient and wild-type strains with nitrite (7.2 mM) provided as the electron acceptor and either acetate (8.6 mM), glucose (3.0 mM), or methanol (11.5 mM) as electron donor (Fig. 2). Both the wild-type and nosZ-deficient strains grown in the presence of all three electron donors reduced almost all of the nitrite within 16 hours, demonstrating that deletion of the nosZ gene did not impair growth. Although nitrite reduction efficiencies were similar in both strains, significantly more nitrous oxide accumulated in the nosZ-deficient cultures. Almost undetectable levels (<0.0006 mmol) of N2O were detected in wild-type cultures, while >0.11 mmol N2O was detected in nosZ-deficient cultures grown on all three electron donors. The N2O conversion efficiencies of the nosZ-deficient mutant grown under all conditions were higher than 60% compared to <0.6% in the wild-type cultures.
Growth of wild-type and nosZ-deficient mutant strains of P. aeruginosa with acetate (8.6 mM), glucose (3.0 mM), or methanol (11.5 mM) provided as electron donors and nitrite (7.2 mM) as electron acceptor. Decreases in nitrite and chemical oxygen demand (COD) concentrations and accumulation of total nitrous oxide (from liquid and gaseous phases) were monitored over time. Each point represents the average of triplicate samples.
When the nosZ-deficient mutant was grown in the presence of acetate and methanol, 81–85% of the NO2− provided as electron acceptor was reduced to N2O (Fig. 3). The mutant strain only reduced 62% of the provided NO2− to N2O when it was grown with glucose provided as the electron donor. It is possible that some of the electrons from glucose were being shuttled into a fermentative pathway30,31 which would explain the lower yield observed with this substrate. However, further investigation into this possibility is required.
Transcription of nitrogen metabolism genes by the nosZ-deletion mutant strain
Quantitative reverse transcription-PCR (qRT-PCR) was conducted with primers targeting denitrification genes (Fig. 1) in order to determine whether deletion of nosZ influenced transcription of other genes in the pathway. The number of mRNA transcripts from norB, the gene coding for the cytochrome c subunit of nitric oxide reductase, was 3.1-fold higher in nosZ-deletion strains than it was in wild-type strains (Fig. 4). Genes coding for nitrite reductase (nirS) and nitrate reductase (narG) were also more significantly expressed in nosZ-deficient strains. These results indicate that deletion of nosZ enhances transcription of genes from nar, nir, and nor operons. It has already been shown that the repression of transcription of nor, nir, and nar genes by the repressor protein Dnr is lifted by NO32,33. It is possible that N2O also helps induce transcription of nitrate, nitrite and nitrous oxide reductases as has been proposed in Rhodobacter sphaeroides34. In addition to induction of the nar promoter by NO, transcription of genes from the nar operon can also be induced by the presence of nitrate32. Although nitrate was never added to the cultures, low concentrations of nitrate (~0.5 mM) were abiotically formed in both the wild-type and nosZ-deficient cultures.
The number of napA, narG, nirB, nirS, norB, and nosZ mRNA transcripts normalized against the number of proC mRNA transcripts detected in wild-type and nosZ-deletion mutant strains of P. aeruginosa grown with acetate (8.6 mM) provided as the electron donor and nitrite (7.2 mM) as the acceptor. Error bars were calculated from triplicate samples.
No transcripts for genes that code for periplasmic nitrate reductase (napA) and the large subunit from assimilatory nitrite reductase (nirB) were detected in either the wild-type or the nosZ-deletion mutant cultures. These results are consistent with previous studies that have shown that napAB is expressed under aerobic and microaerophilic conditions35,36, and that nirB transcription is only induced under high nitrate concentrations37.
Performance of nosZ-deficient mutant strain in a continuous flow partial denitrification bioreactor treating synthetic wastewater
Although pure culture studies demonstrated that the nosZ-deficient strain generated significantly more N2O than the wild-type strain, it was necessary to determine 1) whether the nosZ deletion mutant strain would behave in a similar manner during growth in long-term continuous flow bioreactors, and 2) whether N2O gas generated by this strain during denitrification of high strength total nitrogen wastewater could be efficiently recovered from the bioreactor.
Two denitrification MBBRs treating synthetic anaerobically-treated wastewater with nitrite as the electron acceptor and sole nitrogen source and acetate as the electron donor were operated for 55 days. One of these reactors was bio-augmented with the nosZ-deficient mutant strain (the experimental reactor) and the other reactor was inoculated with the wild-type strain (the control reactor). Throughout the experiment, the nitrogen loading rate was maintained at 0.2~0.3 kgN/(m3 d) to ensure that the denitrification working load was not too high, and the COD/N ratio was maintained at 5.0 to ensure that sufficient electron donor was available for denitrification. Nitrogen removal was >90% in both reactors (Fig. 5), indicating that they were operating stably and efficiently.
During the first stage of the experiment (Stage I: days 1–9), NO2−-N concentrations ranged from 100 to 300 mg/L and COD concentrations ranged from 500 to 1500 mg/L (7.9 to 23.8 mM acetate) (Fig. 5). Little N2O (0.04~0.21 mmol/d) was detected in the control reactor during Stage I. However, approximately 1.1 mmol/d and 5.5 mmol/d of N2O were generated by the experimental reactor when it was provided with NO2−-N concentrations of 100 mg/L and 300 mg/L, respectively. The majority (>97%, Fig. 5a) of N2O being generated in the experimental reactor during Stage I was associated with the dissolved liquid phase, and N2O gas only accounted for 3.6–5.0% of the biogas (Fig. 5). The daily N2O conversion efficiency from NO2−-N was 34% in the experimental reactor, nearly 50 times higher than the conversion efficiency from the control reactor (0.7%).
On day 10 (Stage II: days 10–42), NO2−-N influent concentrations were increased to 500 mg/L, COD was increased to ~2500 mg/L (39.6 mM acetate) and the hydraulic retention time (HRT) was increased from 1 day to 2.25 days. N2O production by the experimental reactor decreased slightly to ~4.5 mmol/d during this stage (Fig. 5) and the majority of N2O was still found in the liquid phase (90–92%). N2O accounted for a higher proportion of biogas (5.5–9.1%) than Stage I (Fig. 6), and the daily N2O conversion efficiency from NO2−-N increased to ~41%. In the control reactor only ~0.5 mmol N2O was produced per day and 4.7% of the removed NO2−-N was converted to N2O.
In the final stage of the experiment (Stage III: days 43–55), influent NO2−-N and COD concentrations were increased to 700 mg/L and ~3500 mg/L (55.4 mM acetate), and HRT was increased to 3 days (Fig. 5). In the experimental reactor, N2O production rates increased to >9 mmol/d and N2O accounted for 73–81% of the biogas (over 200 ml/d) being generated by the reactor. The N2O conversion efficiency also increased during Stage III to over 73% (Fig. 6). In the control reactor, on the other hand, N2O production was still low (<1.7 mmol/d) and the N2O conversion efficiency was <14%.
Discussion
These results clearly showed that bioaugmentation of simple reactors with nosZ-deficient mutant strains of P. aeruginosa significantly improved N2O production. While N2O conversion efficiencies of nosZ-mutant amended reactors reached >73%, previously reported partial nitrification/denitrification reactors operated in a similar manner yielded N2O conversion efficiencies of only 0.8∼7.2%19,38,39. Previous studies controlled operational parameters to enhance N2O conversion efficiencies without considering the fact that much of the N2O was reduced to N2 during the final step of the denitrification pathway. However, by eliminating the final step through deletion of the nosZ gene, we ensured that much of the N2O remained in the reactor system, and this allowed us to achieve significantly higher N2O conversion efficiencies.
Although reactors treating synthetic wastewater with the CANDO approach had conversion efficiencies of 60–65%9, which were still lower than our results, this approach required supplementation of reactors with pulses of electron donor (acetate) and electron acceptor (NO2−) during the denitrification stage which is not always feasible for all wastewater treatment systems. In addition, the need to dose with only acetate during CANDO limits its treatment of actual wastewater with complex organic matter. P. aeruginosa, on the other hand, can utilize a variety of organic compounds found in wastewater as a source of electrons for denitrification40, and high N2O conversion efficiencies (62–85%) were obtained by the nosZ-deficient strain when it was provided with three different electron donors (acetate, methanol, and glucose).
Nitrite concentration and HRT in the experimental reactor appeared to have a significant impact on N2O conversion efficiencies and the proportion of N2O found in the biogas. For example, when influent NO2−-N was lower than 500 mg/L with an HRT of 1.0 d or 2.25 d, the N2O conversion efficiency was 34–41% and N2O only accounted for <6% of the biogas. However, when the influent NO2−-N concentration was increased to 700 mg/L with an HRT of 3.0 d, N2O conversion efficiencies increased to >73% and 73–81% of the biogas was composed of N2O. Higher nitrite concentrations resulted in a higher production of N2O, which became saturated in the aqueous phase and readily emitted into the gaseous phase, thereby increasing N2O biogas content.
The effect of HRT on N2O production and conversion can be explained by the fact that, similar to other denitrification bioreactors, the reactors in this study were operated under anoxic conditions. The feedstock (synthetic wastewater) contained some dissolved oxygen, which competes with nitrite as an electron acceptor and decreases N2O production and conversion5. When the HRT was short (i.e. 1.0 d), the effects of dissolved oxygen on denitrification and N2O production were more significant because of the relatively faster feeding speed. However, when the HRT was prolonged to 3.0 d at Stage III, dissolved oxygen was fed to the reactor at a much slower rate making conditions less oxic and more amenable to denitrification. This might also help explain the finding that N2O conversion rates were consistently lower in bioreactors being continuously fed anoxic wastewater than in pure culture studies carried out in anaerobic serum bottles.
According to eqn. 1, there were significant differences in energy recovery between the experimental and control reactors at all four stages of the experiment when synthetic wastewater contained 100, 300, 500, or 700 mg/L of NO2−-N (Fig. 7). While the experimental reactor amended with the nosZ-deficient mutant strain recovered 64, 298, 552 and 1260 kJ/m3 of energy from N2O, only 2.0, 5.9, 61 and 220 kJ/m3 were recovered from the control reactor. These results showed that throughout operation, energy yields were 5.7~50 times higher in the experimental than the control reactor.
Energy recovery from N2O generated by the experimental reactor (bioaugmented with the nosZ-deficient mutant strain of P. aeruginosa) and the control reactor (bioaugmented with the wild-type strain of P. aeruginosa) when reactors were fed four different influent nitrite concentrations. Error bars represent standard deviations between operational days from each stage.
These results show great promise as a way to recover N2O as an energy source during nitrogen removal processes conducted on high-strength nitrogen wastewater such as leachate41. However, application to real-world treatment strategies needs to be evaluated in further studies.
Methods
Bacterial strains, plasmid and pure culture conditions
Pseudomonas aeruginosa PAO1 was obtained from Dr. Yihe Ge’s laboratory culture collection42. Pseudomonas cells were grown under anaerobic conditions at 37 °C in 50 ml modified AB minimal media43 with 8.6 mM acetate, 11.5 mM methanol or 3.0 mM glucose as electron donor (to theoretically provide the equal COD and electrons) and 7.2 mM NO2−-N (100 mg/L-N) as the electron acceptor for denitrification. All 50 ml cultures were inoculated with 1 ml of P. aeruginosa cells; ~2.5 × 108 cells which had a biomass of 2.54 mg/ml.
Escherichia coli DH-5α and E. coli S17.1 cells were grown in LB media at 37 °C. If appropriate, gentamycin was added at final concentrations of 15 µg/ml for E. coli and 30 µg/ml for P. aeruginosa. The suicide plasmid used in this study was pEXG244.
Construction of nosZ-deletion mutant of Pseudomonas aeruginosa PAO1
The sequences of all primer pairs used for construction of the nosZ deletion mutant are listed in Supplementary Table S1. Primer pairs were designed to amplify regions approximately 500 bp upstream and downstream of the nosZ gene in P. aeruginosa PAO1. PCR was used to add BamHI (GGATCC) and AvrII (CCTAGG) restriction sites to the 5′ and 3′ regions of the nosZ upstream fragment and HindIII (AAGCTT) and AvrII to the 5′ and 3′ regions of the nosZ downstream fragment. PCR products were digested with their appropriate restriction endonucleases (NEB, Beverly, MA), and ligated into the suicide vector pEXG2, resulting in the formation of pEXG2up5′ + 3′dn. Sanger sequencing was done to verify the sequence of the cloned product. A gentamycin resistance cassette was amplified via PCR with flanking AvrII restriction sites using the pBSL141 plasmid as a template. After digestion of both the gentamycin cassette and the pEXG2up5′ + 3′dn plasmid with AvrII, the gentamycin cassette was ligated into the pEXG2up5′ + 3′dn plasmid.
Following plasmid construction, the pEXG2up5′+ gentamycin cassette +3′dn plasmid was chemically transformed into E. coli S17.1 cells and grown on LB agar plates supplemented with 15 µg/ml gentamycin.
Conjugation was then used to transfer the recombinant pEXG2 plasmid into P. aeruginosa PAO1 according to previously described methods45. Deletion of the nosZ gene from the P. aeruginosa chromosome was verified by sequencing (see Supplementary Figs S1, S2).
RNA extraction
RNA was prepared from triplicate cultures of the P. aeruginosa PAO1 nosZ-deletion mutant and wild-type strains grown in modified AB minimal media with 8.6 mM acetate as the electron donor and 7.2 mM NO2− as the electron acceptor. All solutions used during the RNA extraction process were prepared with DEPC-treated water (Adil). Cells were split into 50-ml conical tubes (BD Biosciences, San Jose, CA) and pelleted by centrifugation at 3,000 g for 15 min. RNA was then extracted with the RNAprep Bacteria kit (TianGen, China) and treated with DNA-free DNase (Ambion) according to the manufacturer’s instructions. All RNA samples were checked for integrity by agarose gel electrophoresis and had A260/A280 ratios of 1.8 to 2.2. In order to ensure that RNA samples were not contaminated with DNA, PCR amplification with primers targeting the 16S rRNA gene was conducted on RNA samples that had not undergone reverse transcription.
Quantitative RT-PCR analysis of denitrification pathway genes
Complementary DNA (cDNA) was generated from total RNA (1 μg) extracted from P. aeruginosa PAO1 cells with the FastQuant reverse transcriptase Kit (TianGen, China) according to the manufacturer’s instructions. This cDNA was then used as a template for various PCRs. Sequence data obtained from the DOE Joint Genome Institute (JGI) website (www.jgi.doe.gov) were used to design qRT-PCR primers. All qRT-PCR primers (See Supplementary Table S1) were designed according to the manufacturer’s specifications (amplicon sizes of 100 to 200 bp), and representative products from each of these primer sets were verified by sequencing. qRT-PCR amplification and detection were performed with the 7500 real-time PCR system (Applied Biosystems). Optimal qRT-PCR conditions were determined using the manufacturer’s guidelines. Each PCR mixture consisted of a total volume of 25 µL and contained 1.5 µL of the appropriate primers (stock concentrations, 15 µM) and 12.5 µL SYBR green PCR master mix (Bio-Rad).
Lab-scale denitrification bioreactor set-up
Two 1.5 L lab-scale anaerobic MBBRs (Φ8.0 cm × 30.0 cm) were set up to carry out the partial denitrification treatment of synthetic wastewater with acetate as the electron donor and nitrite as the electron acceptor. NO2−-N concentrations were stepwise increased from 100 mg/L to 700 mg/L, and the COD/N ratio was maintained at 5.0 for the entire period of operation. Each liter of synthetic wastewater also contained 1.0 g K2HPO4, 0.025 g CaCl2, 0.0125 g MgCl2 and 1 mL of trace element solution9. The pH of the synthetic wastewater was adjusted to 8.0 to avoid inhibition of denitrification caused by free nitrous acid46.
One reactor (the experimental reactor) was inoculated with the nosZ-deficient mutant strain of P. aeruginosa, and the other reactor (the control reactor) was inoculated with the wild-type strain of P. aeruginosa PAO1. Both reactors were filled with high-density polyethylene bio-carriers (60% vol). The bio-carriers provided a large surface area for microorganism colonization (Φ7.0 mm × 9.0 mm, specific surface area 900 m2/m3). Two reactors were operated at 37 ± 1 °C during the entire period, and the HRT was stepwise increased from 1.0 day to 3.0 days as the nitrogen loading increased to maintain the nitrogen loading rate at 0.2~0.3 kgN/(m3 d). The biomass concentration in the bioreactor was in the range of 0.7–2.1 gMLVSS/L. The influent/effluent and biogas were sampled every 24 h.
Analytical methods
COD, NO2−-N, NO3−-N and NH4+-N were determined by standard methods47. Cell growth was determined with a spectrophotometer (UV-1800, Shimadzu, Japan) set at a wavelength of 600 nm (OD600). pH was measured with a HACH sensION+ pH3® pH meter (HACH, USA). Oxidation–reduction potential (ORP) was measured with an ORP Meter (M 300, Mettler Toledo, Greifensee, Switzerland) equipped with a Redox Electrode. The ORP of the reactors were maintained at <0 mV throughout the operational periods. Organic acids were measured with high-performance liquid chromatography (HPLC) as previously described48. Total biogas volume in the 2 L-gas-sampling bag was monitored every 24 h with a digital mass flow meter (FMA4000; Omega; USA). The N2O portion of biogas was analyzed with a gas chromatograph (Agilent 7890 A) equipped with an electron capture detector (ECD). N2O dissolved in the liquid phase was calculated with Henrys law as previously described49. Statistical differences were determined by one-way analysis of variance (ANOVA) with p < 0.05 considered to be statistically significant.
Data availability
All data generated or analysed during this study are included in this published article (and its supplementary information files) and are available from the corresponding author on reasonable request.
References
Eggleston, S., Buendia, L. & Miwa, K. 2006 IPCC guidelines for national greenhouse gas inventories [recurso electrónico]: waste. (Kanagawa, J. P.: Institute for Global Environmental Strategies, 2006).
Mosier, A. In International Workshop on Reducing N_2O emissions from Agroecosystems, 1999. 3–15 (Alberta Agriculture Food and Rural Development).
Kroeze, C., Mosier, A. & Bouwman, L. Closing the global N2O budget: a retrospective analysis 1500–1994. Global Biogeochem. Cycles 13, 1–8 (1999).
Law, Y., Ye, L., Pan, Y. & Yuan, Z. Nitrous oxide emissions from wastewater treatment processes. Phil. Trans. R. Soc. B 367, 1265–1277 (2012).
Itokawa, H., Hanaki, K. & Matsuo, T. Nitrous oxide production in high-loading biological nitrogen removal process under low COD/N ratio condition. Water Res. 35, 657–664 (2001).
Tallec, G., Garnier, J., Billen, G. & Gousailles, M. Nitrous oxide emissions from secondary activated sludge in nitrifying conditions of urban wastewater treatment plants: effect of oxygenation level. Water Res. 40, 2972–2980 (2006).
Bock, E. & Wagner, M. In The prokaryotes 457–495 (Springer, 2006).
Todt, D. & Dörsch, P. Mechanism leading to N2O production in wastewater treating biofilm systems. Reviews in Environmental Science and Bio/Technology 15, 355–378 (2016).
Scherson, Y. D. et al. Nitrogen removal with energy recovery through N2O decomposition. Energy & Environmental Science 6, 241–248 (2013).
Scherson, Y. D., Woo, S.-G. & Criddle, C. S. Production of nitrous oxide from anaerobic digester centrate and its use as a co-oxidant of biogas to enhance energy recovery. Environmental science & technology 48, 5612–5619 (2014).
Kampschreur, M. J., Temmink, H., Kleerebezem, R., Jetten, M. S. & van Loosdrecht, M. C. Nitrous oxide emission during wastewater treatment. Water Res. 43, 4093–4103 (2009).
Rassamee, V., Sattayatewa, C., Pagilla, K. & Chandran, K. Effect of oxic and anoxic conditions on nitrous oxide emissions from nitrification and denitrification processes. Biotechnology and Bioengineering 108, 2036–2045 (2011).
Chen, Y. et al. Effects of dissolved oxygen on extracellular enzymes activities and transformation of carbon sources from plant biomass: implications for denitrification in constructed wetlands. Bioresour. Technol. 102, 2433–2440 (2011).
Hanaki, K., Hong, Z. & Matsuo, T. Production of nitrous oxide gas during denitrification of wastewater. Water Sci. Technol. 26, 1027–1036 (1992).
Schulthess, R., Kühni, M. & Gujer, W. Release of nitric and nitrous oxides from denitrifying activated sludge. Water Res. 29, 215–226 (1995).
Park, K. Y., Inamori, Y., Mizuochi, M. & Ahn, K. H. Emission and control of nitrous oxide from a biological wastewater treatment system with intermittent aeration. J. Biosci. Bioeng. 90, 247–252 (2000).
Tsuneda, S., Mikami, M., Kimochi, Y. & Hirata, A. Effect of salinity on nitrous oxide emission in the biological nitrogen removal process for industrial wastewater. J. Hazard. Mater. 119, 93–98 (2005).
Lemaire, R. et al. Identifying causes for N2O accumulation in a lab-scale sequencing batch reactor performing simultaneous nitrification, denitrification and phosphorus removal. J. Biotechnol. 122, 62–72 (2006).
Kampschreur, M. J. et al. Dynamics of nitric oxide and nitrous oxide emission during full-scale reject water treatment. Water Research 42, 812–826 (2008).
Weiss, R. & Price, B. Nitrous oxide solubility in water and seawater. Marine chemistry 8, 347–359 (1980).
Chen, M.-Y. et al. Pseudoxanthomonas taiwanensis sp. nov., a novel thermophilic, N2O-producing species isolated from hot springs. International journal of systematic and evolutionary microbiology 52, 2155–2161 (2002).
Finkmann, W., Altendorf, K., Stackebrandt, E. & Lipski, A. Characterization of N2O-producing Xanthomonas-like isolates from biofilters as Stenotrophomonas nitritireducens sp. nov., Luteimonas mephitis gen. nov., sp. nov. and Pseudoxanthomonas broegbernensis gen. nov., sp. nov. International journal of systematic and evolutionary microbiology 50, 273–282 (2000).
Hart, L. T., Larson, A. & McCleskey, C. Denitrification by Corynebacterium nephridii. Journal of bacteriology 89, 1104–1108 (1965).
Wang, W. et al. Identification of a repressor of a truncated denitrification pathway in Moraxella catarrhalis. J. Bacteriol. 190, 7762–7772 (2008).
Wang, X., Hu, M., Xia, Y., Wen, X. & Ding, K. Pyrosequencing analysis of bacterial diversity in 14 wastewater treatment systems in China. Applied and environmental microbiology 78, 7042–7047 (2012).
Zhang, T., Shao, M.-F. & Ye, L. 454 Pyrosequencing reveals bacterial diversity of activated sludge from 14 sewage treatment plants. The ISME journal 6, 1137 (2012).
Waheed, H., Hashmi, I., Naveed, A. K. & Khan, S. J. Molecular detection of microbial community in a nitrifying–denitrifying activated sludge system. International Biodeterioration & Biodegradation 85, 527–532 (2013).
Szekeres, S., Kiss, I., Kalman, M. & Soares, M. I. M. Microbial population in a hydrogen-dependent denitrification reactor. Water research 36, 4088–4094 (2002).
Borrero-de Acuña, J. M. et al. Protein network of the Pseudomonas aeruginosa denitrification apparatus. Journal of bacteriology 198, 1401–1413 (2016).
Schreiber, K. et al. Anaerobic survival of Pseudomonas aeruginosa by pyruvate fermentation requires an Usp-type stress protein. Journal of bacteriology 188, 659–668 (2006).
Eschbach, M. et al. Long-term anaerobic survival of the opportunistic pathogen Pseudomonas aeruginosa via pyruvate fermentation. Journal of bacteriology 186, 4596–4604 (2004).
Schreiber, K. et al. The anaerobic regulatory network required for Pseudomonas aeruginosa nitrate respiration. Journal of bacteriology 189, 4310–4314 (2007).
Spiro, S. Nitrous oxide production and consumption: regulation of gene expression by gas-sensitive transcription factors. Philosophical Transactions of the Royal Society B: Biological Sciences 367, 1213–1225 (2012).
Sabaty, M., Schwintner, C., Cahors, S., Richaud, P. & Verméglio, A. Nitrite and nitrous oxide reductase regulation by nitrogen oxides in Rhodobacter sphaeroides f. sp. denitrificans IL106. Journal of bacteriology 181, 6028–6032 (1999).
Potter, L., Angove, H., Richardson, D. & Cole, J. Nitrate reduction in the periplasm of gram-negative bacteria (2001).
Van Alst, N. E., Sherrill, L. A., Iglewski, B. H. & Haidaris, C. G. Compensatory periplasmic nitrate reductase activity supports anaerobic growth of Pseudomonas aeruginosa PAO1 in the absence of membrane nitrate reductase. Canadian journal of microbiology 55, 1133–1144 (2009).
Wang, H. & Gunsalus, R. P. The nrfA and nirB nitrite reductase operons in Escherichia coli are expressed differently in response to nitrate than to nitrite. Journal of bacteriology 182, 5813–5822 (2000).
Ahn, J. H., Kwan, T. & Chandran, K. Comparison of partial and full nitrification processes applied for treating high-strength nitrogen wastewaters: microbial ecology through nitrous oxide production. Environmental science & technology 45, 2734–2740 (2011).
Foley, J., De Haas, D., Yuan, Z. & Lant, P. Nitrous oxide generation in full-scale biological nutrient removal wastewater treatment plants. Water research 44, 831–844 (2010).
Firestone, M. Biological denitrification. Nitrogen in agricultural soils, 289–326 (1982).
Liu, Z., Dang, Y., Li, C. & Sun, D. Inhibitory effect of high NH4+-N concentration on anaerobic biotreatment of fresh leachate from a municipal solid waste incineration plant. Waste Management 43, 188–195 (2015).
Cui, Q. et al. Cross-Regulation between the phz1 and phz2 operons maintain a balanced level of phenazine biosynthesis in Pseudomonas aeruginosa PAO1. PloS one 11, e0144447 (2016).
Heydorn, A. et al. Quantification of biofilm structures by the novel computer program COMSTAT. Microbiology 146, 2395–2407 (2000).
Rietsch, A., Vallet-Gely, I., Dove, S. L. & Mekalanos, J. J. ExsE, a secreted regulator of type III secretion genes in Pseudomonas aeruginosa. Proceedings of the National Academy of Sciences 102, 8006–8011 (2005).
Hmelo, L. R. et al. Precision-engineering the Pseudomonas aeruginosa genome with two-step allelic exchange. Nature protocols 10, 1820 (2015).
Zhou, Y., Pijuan, M., Zeng, R. J. & Yuan, Z. Free nitrous acid inhibition on nitrous oxide reduction by a denitrifying-enhanced biological phosphorus removal sludge. Environ. Sci. Technol. 42, 8260–8265 (2008).
APHA, A., WPCF. Standard Methods for Water and Wastewater Examination,twenty-firsted. Vol. 2 (APHA, Washington, DC, USA., 2005).
Dang, Y. et al. Impact of fulvic acids on bio-methanogenic treatment of municipal solid waste incineration leachate. Water Research 106, 71–78 (2016).
Moraghan, J. & Buresh, R. Correction for Dissolved Nitrous Oxide in NitrogenStudies 1. Soil Sci. Soc. Am. J. 41, 1201–1202 (1977).
Acknowledgements
This research was financially supported by the National Natural Science Foundation of China (51678051).
Author information
Authors and Affiliations
Contributions
D.S. and Y.D. conceived the experiments; Z.L. performed the experiments with help from Y.D. and D.E.H., D.E.H., Y.D. and Z.L. analyzed the data and wrote the manuscript. All authors have seen the manuscript at all stages, discussed the data and agreed to the content.
Corresponding authors
Ethics declarations
Competing Interests
The authors declare no competing interests.
Additional information
Publisher's note: Springer Nature remains neutral with regard to jurisdictional claims in published maps and institutional affiliations.
Electronic supplementary material
Rights and permissions
Open Access This article is licensed under a Creative Commons Attribution 4.0 International License, which permits use, sharing, adaptation, distribution and reproduction in any medium or format, as long as you give appropriate credit to the original author(s) and the source, provide a link to the Creative Commons license, and indicate if changes were made. The images or other third party material in this article are included in the article’s Creative Commons license, unless indicated otherwise in a credit line to the material. If material is not included in the article’s Creative Commons license and your intended use is not permitted by statutory regulation or exceeds the permitted use, you will need to obtain permission directly from the copyright holder. To view a copy of this license, visit http://creativecommons.org/licenses/by/4.0/.
About this article
Cite this article
Lin, Z., Sun, D., Dang, Y. et al. Significant enhancement of nitrous oxide energy yields from wastewater achieved by bioaugmentation with a recombinant strain of Pseudomonas aeruginosa. Sci Rep 8, 11916 (2018). https://doi.org/10.1038/s41598-018-30326-8
Received:
Accepted:
Published:
DOI: https://doi.org/10.1038/s41598-018-30326-8
- Springer Nature Limited