Abstract
Temperature and strain rate related dynamic recrystallization (DRX) and its inherited effects on ductile fracture have become an urgent issue which impede accurate prediction of fracture strain and restrict formability for 7075 aluminum alloy in hot deformation process. In order to precisely elaborate the ductile fracture behavior of 7075 aluminum alloy during hot forming process and accurately predict the initiation of ductile fracture, an extended ductile fracture criterion (DFC) at elevated temperature was established considering DRX effects and various stress states. The relationship between fracture strain and Z parameter is revealed in DRX and DRX-free region, respectively. It is noted that fracture strain decreases with the increasing Z parameter in DRX region, while Z parameter has little effect on the fracture behavior in DRX-free region. Consequently, the Z parameter embedded DRX model is introduced into the modified Mohr–Coulomb (MMC) DFC under distinct stress states at elevated temperatures for 7075 aluminum alloy. Based on the Abaqus/Explicit platform, the proposed ductile fracture model is implemented in finite element simulation via VUMAT. Hot forming of T-shaped parts is carried out, and the predicted fracture scenarios including damage evolution, volume fraction of DRX are validated by experimental results.


























Similar content being viewed by others
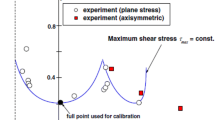
References
Cao TS, Gachet JM, Montmitonnet P et al (2014) A Lode-dependent enhanced Lemaitre model for ductile fracture prediction at low stress triaxiality. Eng Fract Mech 124–125:80–96. https://doi.org/10.1016/j.engfracmech.2014.03.021
Bonora N, Testa G, Ruggiero A et al (2020) Continuum damage mechanics modelling incorporating stress triaxiality effect on ductile damage initiation. Fatigue Eng Mater Struct 43:13220. https://doi.org/10.1111/ffe.13220
Mohr D, Marcadet SJ (2015) Micromechanically-motivated phenomenological Hosford-Coulomb model for predicting ductile fracture initiation at low stress triaxialities. Int J Solids Struct 67–68:40–55. https://doi.org/10.1016/j.ijsolstr.2015.02.024
Ma H, Xu W, Jin BC et al (2015) Damage evaluation in tube spinnability test with ductile fracture criteria. Int J Mech Sci 100:99–111. https://doi.org/10.1016/j.ijmecsci.2015.06.005
Cockcroft MG, Latham DJ (1968) Ductility and the workability of metals. J of Metals 96:2444
Rice JR, Tracey DM (1969) On the ductile enlargement of voids in triaxial stress fields. J Mech Phys Solids 17:201–217. https://doi.org/10.1016/0022-5096(69)90033-7
Xue L (2007) Damage accumulation and fracture initiation in uncracked ductile solids subject to triaxial loading. Int J Solids Struct 44:5163–5181. https://doi.org/10.1016/j.ijsolstr.2006.12.026
Bai Y, Wierzbicki T (2008) A new model of metal plasticity and fracture with pressure and Lode dependence. Int J Plast 24:1071–1096. https://doi.org/10.1016/j.ijplas.2007.09.004
Lou Y, Huh H (2013) Extension of a shear-controlled ductile fracture model considering the stress triaxiality and the Lode parameter. Int J Solids Struct 50:447–455. https://doi.org/10.1016/j.ijsolstr.2012.10.007
Lou Y, Huh H, Lim S et al (2012) New ductile fracture criterion for prediction of fracture forming limit diagrams of sheet metals. Int J Solids Struct 49:3605–3615. https://doi.org/10.1016/j.ijsolstr.2012.02.016
Lou Y, Yoon JW, Huh H (2014) Modeling of shear ductile fracture considering a changeable cut-off value for stress triaxiality. Int J Plasticity 54:56–80. https://doi.org/10.1016/j.ijplas.2013.08.006
Lou Y, Chen L, Clausmeyer T et al (2017) Modeling of ductile fracture from shear to balanced biaxial tension for sheet metals. Int J Solids Struct 112:169–184. https://doi.org/10.1016/j.ijsolstr.2016.11.034
Bai Y, Wierzbicki T (2010) Application of extended Mohr-Coulomb criterion to ductile fracture. Int J Fract 161:1–20. https://doi.org/10.1007/s10704-009-9422-8
Jia Y, Bai Y (2016) Ductile fracture prediction for metal sheets using all-strain-based anisotropic eMMC model. Int J Mech Sci 115–116:516–531. https://doi.org/10.1016/j.ijmecsci.2016.07.022
Jia Y, Bai Y (2016) Experimental study on the mechanical properties of AZ31B-H24 magnesium alloy sheets under various loading conditions. Int J Fract 197:25–48. https://doi.org/10.1007/s10704-015-0057-7
Qian L, Fang G, Zeng P (2017) Modeling of the ductile fracture during the sheet forming of aluminum alloy considering nonassociated constitutive characteristic. Int J Mech Sci 126:55–66. https://doi.org/10.1016/j.ijmecsci.2017.03.013
Tang B, Wang Q, Guo N et al (2020) Modeling anisotropic ductile fracture behavior of Ti-6Al-4V titanium alloy for sheet forming applications at room temperature. Int J Solids Struct 207:178–195. https://doi.org/10.1016/j.ijsolstr.2020.10.011
Qian L, Paredes M, Wierzbicki T et al (2016) Experimental and numerical study on shear-punch test of 6060 T6 extruded aluminum profile. Int J Mech Sci 118:205–218. https://doi.org/10.1016/j.ijmecsci.2016.09.008
Cai W, Jiang J, Li G et al (2021) Fracture behavior of high-strength bolted steel connections at elevated temperatures. Eng Struct 245:112817. https://doi.org/10.1016/j.engstruct.2021.112817
Cai W, Jiang J, Li G (2021) Analysis and simulation on fracture of structural steel at elevated temperatures based on extended finite element method. Fire Saf J 120:103022. https://doi.org/10.1016/j.firesaf.2020.103022
Cai W, Jiang J, Wang Y et al (2020) Fracture behavior of high-strength steels at elevated temperatures. J Constr Steel Res 175:106385. https://doi.org/10.1016/j.jcsr.2020.106385
Kacem A, Laurent H, Thuillier S (2022) Experimental and numerical investigation of ductile fracture for AA6061-T6 sheets at room and elevated temperatures. Int J Mech Sci 222:107201. https://doi.org/10.1016/j.ijmecsci.2022.107201
Tian X, Guo J (2022) Dynamic mechanical properties and modification of fracture criteria of 6061–T651 aluminum alloy. Eng Fract Mech 262:108273. https://doi.org/10.1016/J.ENGFRACMECH.2022.108273
Jia W, Ma L, Jiao M et al (2020) Fracture criterion for predicting edge-cracking in Hot rolling of twin-roll casted AZ31 Mg alloy. J Mater Res Technol 9:4773–4787. https://doi.org/10.1016/j.jmrt.2020.02.103
Ning F, Zhou X, Le Q et al (2020) Fracture and deformation characteristics of AZ31 magnesium alloy plate during tension rolling. Mater Today Commun 24:101129. https://doi.org/10.1016/j.mtcomm.2020.101129
Zhang X, Zeng W, Shu Y et al (2009) Fracture criterion for predicting surface cracking of Ti40 alloy in hot forming processes. T Nonferr Metal Soc 19:5. https://doi.org/10.1016/S1003-6326(08)60263-0
Hashemi SJ, Naeini HM, Liaghat GH et al (2015) Prediction of bulge height in warm hydroforming of aluminum tubes using ductile fracture criteria. Arch Civ Mech Eng 15:19–29. https://doi.org/10.1016/j.acme.2014.08.003
Kim WJ, Kim WY, Kim HK (2010) Hot-air forming of Al-Mg-Cr alloy and prediction of failure based on Zener-Holloman parameter. Met Mater Int 16:895–903. https://doi.org/10.1007/s12540-010-1207-0
Shang X, Cui Z, Fu MW (2018) A ductile fracture model considering stress state and Zener-Hollomon parameter for hot deformation of metallic materials. Int J Mech Sci 144:800–812. https://doi.org/10.1016/j.ijmecsci.2018.06.030
Dehghan-Manshadi A, Barnett MR, Hodgson PD (2008) Hot deformation and recrystallization of austenitic stainless steel: Part I. Dynamic Recrystallization Metall Mater Trans 39:1359–1370. https://doi.org/10.1007/s11661-008-9512-7
Zhang J, Li Z, Wen K et al (2019) Simulation of dynamic recrystallization for an Al-Zn-Mg-Cu alloy using cellular automaton. Prog Nat Sci: Mater Int 29:477–484. https://doi.org/10.1016/j.pnsc.2019.04.002
Wang S, Luo J, Hou L et al (2016) Physically based constitutive analysis and microstructural evolution of AA7050 aluminum alloy during hot compression. Mater Des 107:277–289. https://doi.org/10.1016/j.matdes.2016.06.023
Xiao X, Mu Z, Pan H et al (2018) Effect of the Lode parameter in predicting shear cracking of 2024–T351 aluminum alloy Taylor rods. Int J Impact Eng 120:185–201. https://doi.org/10.1016/j.ijimpeng.2018.06.008
Xiao X, Wang Y, Vershinin VV et al (2019) Effect of Lode angle in predicting the ballistic resistance of Weldox 700 E steel plates struck by blunt projectiles. Int J Impact Eng 128:46–71. https://doi.org/10.1016/j.ijimpeng.2019.02.004
Wang X, Zhang Y, Ma X (2020) High temperature deformation and dynamic recrystallization behavior of AlCrCuFeNi high entropy alloy. Mater Res Express 778:139077. https://doi.org/10.1016/j.msea.2020.139077
Liu J, Wang X, Liu J et al (2019) Hot deformation and dynamic recrystallization behavior of Cu-3Ti-3Ni-0.5Si alloy. J Alloys Compd 782:224–234. https://doi.org/10.1016/j.jallcom.2018.12.212
Ban Y, Zhang Y, Tian B et al (2020) EBSD analysis of hot deformation behavior of Cu-Ni-Co-Si-Cr alloy. Mater Charact 169:110656. https://doi.org/10.1016/j.matchar.2020.110656
Wu S, Liu Z, Huang X et al (2021) Process parameter optimization and EBSD analysis of Ni60A-25% WC laser cladding. Int J Refract Hard Met 101:105675. https://doi.org/10.1016/j.ijrmhm.2021.105675
Dalai B, Moretti MA, Kerstrm P et al (2021) Mechanical behavior and microstructure evolution during deformation of AA7075-T651. Mater Sci Eng A 822:141615
Johnson GR, WH C (1983) A constitutive model and data for materials subjected to large strains, high strain rates, and high temperatures. Eng Fract Mech 541–547.
Hu M, Sun Y, He J et al (2022) Hot deformation behaviour and microstructure evolution of Al-3%Mg2Si alloy. Mater Charact 183:111623. https://doi.org/10.1016/j.matchar.2021.111623
Huang W, Chen J, Zhang R et al (2022) Effect of deformation modes on continuous dynamic recrystallization of extruded AZ31 Mg alloy. J Alloys Compd 897:163086. https://doi.org/10.1016/j.jallcom.2021.163086
Lin X, Huang H, Yuan X et al (2022) Study on high-temperature deformation mechanical behavior and dynamic recrystallization kinetics model of Ti-47.5Al-2.5V-1.0Cr-0.2Zr alloy. J Alloys Compd 891:162105. https://doi.org/10.1016/j.jallcom.2021.162105
Fan H, Jiang H, Dong J et al (2019) An optimization method of upsetting process for homogenized, nickel-based superalloy Udimet 720Li ingot considering both cracking and recrystallization. J Mater Process Technol 269:52–64. https://doi.org/10.1016/j.jmatprotec.2019.01.013
Mofarrehi M, Javidani M, Chen XG (2022) Effect of Mn content on the hot deformation behavior and microstructure evolution of Al–Mg–Mn 5xxx alloys. Mater Res Express 845:143217. https://doi.org/10.1016/j.msea.2022.143217
Lou Y, Huh H (2013) Prediction of ductile fracture for advanced high strength steel with a new criterion: experiments and simulation. J Mater Process Technol 213:1284–1302. https://doi.org/10.1016/j.jmatprotec.2013.03.001
Kacem A, Laurent H, Thuillier S (2021) Influence of experimental boundary conditions on the calibration of a ductile fracture criterion. Eng Fract Mech 248:107686. https://doi.org/10.1016/j.engfracmech.2021.107686
Ebrahimi R, Najafizadeh A (2004) A new method for evaluation of friction in bulk metal forming. J Mater Process Technol 152:136–143. https://doi.org/10.1016/j.jmatprotec.2004.03.029
Wan P, Kang T, Li F et al (2021) Dynamic recrystallization behavior and microstructure evolution of low-density high-strength Fe–Mn–Al–C steel. J Mater Res Technol 15:1059–1068. https://doi.org/10.1016/j.jmrt.2021.08.079
Xu Y, Chen C, Zhang X et al (2018) Dynamic recrystallization kinetics and microstructure evolution of an AZ91D magnesium alloy during hot compression. Mater Charact 145:39–52. https://doi.org/10.1016/j.matchar.2018.08.028
Quan G, Mao Y, Li G et al (2012) A characterization for the dynamic recrystallization kinetics of as-extruded 7075 aluminum alloy based on true stress–strain curves. Comput Mater Sci 55:65–72. https://doi.org/10.1016/j.commatsci.2011.11.031
Tang B, Li H, Guo N et al (2021) Revealing ductile/quasi-cleavage fracture and DRX-affected grain size evolution of AA7075 alloy during hot stamping process. Int J Mech Sci 212:106243. https://doi.org/10.1016/j.ijmecsci.2021.106843
Acknowledgements
Acknowledgement is given to financial support by the National Natural Science Foundation of China (#52105370, #52275344), the Natural Science Foundation of Shandong Province (#ZR2020QE168, #ZR2020KE021), Innovation Team of Jinan (#2019GXRC035), the Pilot Project plan for Integration of Science, Education and Production of Qilu University of Technology (Shandong Academy of Sciences) (#2022PY032, #2022PT007) and Collaborative Innovation Foundation of Qilu University of Technology (Shandong Academy of Sciences) (#2021CXY-05).
Author information
Authors and Affiliations
Corresponding authors
Ethics declarations
Conflicts of interest
The authors declare no conflict of interest.
Additional information
Publisher's note
Springer Nature remains neutral with regard to jurisdictional claims in published maps and institutional affiliations.
Highlights
• An extended ductile fracture criterion at elevated temperature was established considering DRX effects and various stress states.
• Fracture strain decreases with the increasing of Z parameter in DRX region, while little impact in DRX-free region.
• The influence of process parameters on the formability of T-shaped parts are predicted.
• DRX and its inherited effects on ductile fracture have been revealed for AA7075 alloy in hot deformation process.
Appendix A. Z parameter calibration process
Appendix A. Z parameter calibration process
In order to determine the characteristic parameters of critical values and peaks applicable to all flow curves studied for 7075 aluminum alloy, a work hardening rate \(\theta\) (\(\theta =\partial \sigma /\partial \varepsilon\)) \(-\sigma\) curve should be plotted, shown in Fig. 27. The work hardening-stress curves at different temperatures and strain rate are shown in Fig. 28. Using the method proposed in the literature, \({\sigma }_{c}\) is determined as the second derivative of \(\theta\) relative to \(\sigma\), that is, the stress value when \({\partial }^{2}\theta /\partial {\sigma }^{2}\) is 0. The peak stress \({\sigma }_{p}\) is defined as the point at \(\theta =0\). Then, according to the stress–strain curve, the corresponding turning strain \({\varepsilon }_{c}\) and peak strain \({\sigma }_{p}\) are obtained.
The dynamic recovery softening coefficient \(\Omega\) is solved by the equation for the work hardening-dynamic recovery stage before the transition strain:
Starting from Eq. (A.1), the \(\mathrm{ln}\Omega -\mathrm{ln}Z\) relationship diagram is drawn as shown in Fig. 29, and the data in Fig. 29 is fitted, then the mathematical model of \(\Omega\) can be determined as:
The dynamic recrystallization percentage \({X}_{drx}\) is usually expressed as:
where \({X}_{drx}\) is the recrystallization volume fraction, \({k}_{d}\) and \({n}_{d}\) are constants, \({\varepsilon }_{c}\) is the critical strain, and \({\varepsilon }_{p}\) is the peak strain. By fitting the experimentally obtained \(\mathit{ln}\left(-\mathit{ln}\left(1-{X}_{drx}\right)\right)\) and \(ln(\varepsilon -{\varepsilon }_{c}/{\varepsilon }_{p})\), the values of \({k}_{d}\) and \({n}_{d}\) can be determined: \({k}_{d}\)=\(-\) 0.1906, \({n}_{d}\)=1.6121.
Rights and permissions
Springer Nature or its licensor (e.g. a society or other partner) holds exclusive rights to this article under a publishing agreement with the author(s) or other rightsholder(s); author self-archiving of the accepted manuscript version of this article is solely governed by the terms of such publishing agreement and applicable law.
About this article
Cite this article
Tang, B., Li, M., Guo, N. et al. Genetic effects of dynamic recrystallization on ductile fracture at elevated temperature for AA7075 alloy with various stress states: modeling and simulation. Int J Mater Form 16, 9 (2023). https://doi.org/10.1007/s12289-022-01730-3
Received:
Accepted:
Published:
DOI: https://doi.org/10.1007/s12289-022-01730-3