Abstract
Background
Several factors have been shown to contribute to hypoxic-induced declined in aerobic capacity. In the present study, we investigated the effects of resting hypoxic ventilatory and cardiac responses (HVR and HCR) on hypoxic-induced declines in peak oxygen uptake (\(\dot{\mathrm V}\)O2peak).
Methods
Peak oxygen uptakes was measured in normobaric normoxia (room air) and hypoxia (14.1% O2) for 10 young healthy men. The resting HVR and HCR were evaluated at multiple steps of hypoxia (1 h at each of 21, 18, 15 and 12% O2). Arterial desaturation (ΔSaO2) was calculate by the difference between SaO2 at normoxia—at each level of hypoxia (%). HVR was calculate by differences in pulmonary ventilation between normoxia and each level of hypoxia against ΔSaO2 (L min−1 %−1 kg−1). Similarly, HCR was calculated by differences in heart rate between normoxia and each level of hypoxia against ΔSaO2 (beats min−1 %−1).
Results
\(\dot{\mathrm V}\)O2peak significantly decreased in hypoxia by 21% on average (P < 0.001). HVR was not associated with changes in \(\dot{\mathrm V}\)O2peak. ΔSaO2 from normoxia to 18% or 15% O2 and HCR between normoxia and 12% O2 were associated with changes in \(\dot{\mathrm V}\)O2peak (P < 0.05, respectively). The most optimal model using multiple linear regression analysis found that ΔHCR at 12% O2 and ΔSaO2 at 15% O2 were explanatory variables (adjusted R2 = 0.580, P = 0.02).
Conclusion
These results suggest that arterial desaturation at moderate hypoxia and heart rate responses at severe hypoxia may account for hypoxic-induced declines in peak aerobic capacity, but ventilatory responses may be unrelated.
Similar content being viewed by others
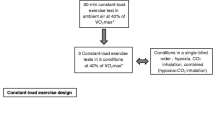
Background
Recently, there have been an increase in the numbers of individuals visiting high-altitude locations for work, mountaineering, skiing, or exercise training. Maintaining physical performance, including aerobic capacity, at high altitude may be important for maximizing training adaptation and preventing accidents.
However, it is well known that peak aerobic capacity (i.e., peak oxygen uptake; \(\dot{\mathrm V}\)O2peak) progressively declines with increasing altitude [1]. Since \(\dot{\mathrm V}\)O2 during moderate-intensity exercise is similar between normoxia and hypoxia ~12% O2 [2, 3], a decline in \(\dot{\mathrm V}\)O2peak at high altitude may affect submaximal exercise performance. Nonetheless, the underlying mechanism(s) causing the decline in \(\dot{\mathrm V}\)O2peak at high altitude are controversial and have no universal consensus.
Hypoxic ventilatory responses (HVRs) can explain the reductions in \(\dot{\mathrm V}\)O2peak in hypoxia [4, 5]; however, another study disagreed [6]. An important point is that individual-related variability in response to hypoxic exposure may relate to hypoxic-induced changes in peak aerobic capacity [6]. Moreover, these studies evaluated only respiratory responses to hypoxia [4,5,6] without evaluation of hypoxic cardiac responses to account for declines in \(\dot{\mathrm V}\)O2peak. Previous studies assessed HVR at only one level of hypoxia [5, 6]. To our knowledge, no studies evaluated HVRs at multiple steps of hypoxia against changes in peak aerobic capacity. Additionally, increasing altitude decreased \(\dot{\mathrm V}\)O2peak [1] and blunted hypoxic cardiac responses (HCR) [7]; however, to the best of our knowledge, no studies have examined about a relationship between changes in \(\dot{\mathrm V}\)O2peak and HCR even at one level of hypoxia. Thus, the level of hypoxia and resting hypoxic ventilatory and/or cardiac responses are necessary to exert hypoxic-induced changes in peak aerobic capacity effects. A previous study reported that climbers who maintained their arterial oxygen saturation level from an altitude of 2400 m to 5300 m did not develop symptoms of acute mountain sickness [8]. Thus, considering about actual site, progressive declines in oxygen level protocol may be relevant because it could simulate actual mountain climbing such as from sea level to gradual ascent.
This study therefore aimed to evaluate resting hypoxic respiratory and circulatory responses at multiple steps of hypoxia and to explain hypoxic-induced declines in \(\dot{\mathrm V}\)O2peak. We hypothesized that resting hypoxic respiratory and/or circulatory responses may account for declines in \(\dot{\mathrm V}\)O2peak in hypoxia and that these responses would change with different oxygen levels.
Method
Participants
This study used additional data from already published investigations [9] from an entirely different perspective, which was approved by the ethical committee of the Mount Fuji Research Institute in Japan and performed in accordance with the guidelines of the Declaration of Helsinki. Ten healthy male lowlanders were involved with a mean age of 23 ± 2 years, height of 174.3 ± 7.8 cm, and body weight of 72.4 ± 16.7 kg (mean ± standard deviation). The participants did not engage in regular exercise. They were free from any known cardiorespiratory and cardiovascular diseases and had not taken any medications. Since a previous study demonstrated that previous intermittent hypoxic exposure influences hypoxic ventilatory and cardiovascular responses [10], none of the participants was exposed to an altitude higher than 1500 m within 6 months before the study in accordance with a previous study [11]. Participants were requested to abstain from caffeinated beverages for 12 h and from strenuous physical activity and alcohol for at least 24 h before the study. They were familiarized with all measurement techniques, i.e., cycling exercise at 60 revolutions per minute, hypoxic exposure with a face mask. All participants signed an informed consent form.
Procedures
The study comprised three experimental protocols: determination of \(\dot{\mathrm V}\)O2peak (i) in normoxia, (ii) in hypoxia and (iii) in progressive normobaric hypoxia. All studies were performed at an ambient temperature of 24 ± 1°C.
A determination of individual \(\dot{\mathrm V}\)O2peak was performed using a bicycle ergometer (Monark 828E; Monark, Vansbro, Sweden) while inspiring room air (normobaric normoxia) or 14.1% O2 (normobaric hypoxia). This inspired oxygen fraction is equivalent to an altitude of 3200 m at which a large drop in oxygen saturation is observed with a small drop in pressure of O2 [12], which in turn acts as a stimulator to enhance exercise performance in many athletes engaging in hypoxic training [13]. Participants performed an incremental leg cycling test with an increasing rate of 30 watts per minute at 60 revolutions per minute in an upright position until exhaustion. The criteria for exhaustion were as follows: (i) no increase in oxygen uptake, despite a further increase in work rate; (ii) heart rate 95% of age-predicted maximal values (220—age); (iii) rating of perceived exertion reaching 19; or (iv) failure to maintain pedaling frequency of 60 revolutions per minute despite a strong verbal encouragement. The test was terminated when at least two of the above four criteria were met [14]. The order of these two tests was randomized and counterbalanced.
For the progressive hypoxic exposure test, each participant sat on a comfortable chair in a semi-recumbent position. They breathed room air (21% O2), followed by three graded hypoxic gas mixtures containing 18, 15, and 12% O2 for 60 min each. The last 5 min during the first 30 min of exposure (i.e., 25–30 min during each exposure period) were recorded (Fig. 1). This is because it has been reported that ventilatory responses was blunted 30 min after hypoxic exposure [15], and other measurements were performed during the latter 30 min in the previous study [9]. The hypoxic gas was continuously blended using a gas mixing system (YHS-B05S; YKS, Nara, Japan) and delivered from a 200 L Douglas bag reservoir through a two-way, non-rebreathing valve and face mask (Fig. 2).
Measurements
Pulmonary ventilation (\(\dot{\mathrm V}\)E; L min−1), \(\dot{\mathrm V}\)O2, and carbon dioxide output (\(\dot{\mathrm V}\)CO2) were continuously measured by an online computerized metabolic cart (aero monitor AE-300S, Minato Medical Science, Osaka, Japan). The \(\dot{\mathrm V}\)E, \(\dot{\mathrm V}\)O2, and \(\dot{\mathrm V}\)CO2 were averaged every 10 s. Heart rate (HR; beats min−1) and peripheral arterial oxygen saturation (SaO2; %) were monitored with a wireless HR monitor (POLAR RC800X, POLAR Electro, Tokyo, Japan) and finger pulse oximetry (PULSOX-300i, Konica Minolta, Tokyo, Japan), respectively. These data (HR and SpO2) were also averaged every 10 s.
Data analysis
Based on previous studies [7, 16], the hypoxic desaturation (ΔSaO2), hypoxic ventilatory (HVR), and cardiac (HCR) responses were calculated by the following equations.
The HVR and HCR were calculated using data every 10 s (see above) and averaged over the 5-min period. Since ΔSaO2 is defined as the difference from normoxia to hypoxia, greater ΔSaO2 values indicate greater arterial desaturation. Accordingly, greater values of HVR or HCR mean more effective responses to hypoxia [7, 16]. Percent changes in \(\dot{\mathrm V}\)O2peak, HVR, and HCR was calculated by a following equation.
Statistical analysis
Values are mean ± standard deviation. A paired t-test was used for \(\dot{\mathrm V}\)O2peak comparison between normoxia and hypoxia. One-way repeated-measures analysis of variance with pairwise (Bonferroni) post hoc tests were used to evaluate the changes in all cardiorespiratory variables (\(\dot{\mathrm V}\)E, HR, SaO2, SaO2, HVR, and HCR) across different oxygen levels. Effect size was calculated as “η2,” defined as small (η2 = 0.01), medium (η2 = 0.06), and large (η2 = 0.14) effects [17]. The Pearson correlation coefficient was used for the relationship between decreases in \(\dot{\mathrm V}\)O2peak and changes in SaO2, HVR, and HCR at each level. When a significant correlation was found between variables, we performed multiple linear regression analysis using variables that was recognized a significant relationship to explain changes in \(\dot{\mathrm V}\)O2peak. Although high variance inflation factors (VIFs > 5) indicate multicollinearity [18], the VIFs were < 3 for all explanatory variables. To identify the optimal model containing the parameters to best explain the data, we performed model selection by backward stepwise elimination using Akaike Information Criterion based on our previous studies [19, 20]. All statistical analyses were performed using R ver. 2.13.1. A P value < 0.05 was considered statistically significant.
Results
Table 1 showed cardio respiratory variables at exhaustion during an incremental exercise test.
\(\dot{\mathrm V}\)O2peak and \(\dot{\mathrm V}\)CO2peak during the incremental leg cycling test decreased in hypoxia compared with that in normoxia (P < 0.001, respectively). The average decrease in \(\dot{\mathrm V}\)O2peak was − 21.4 ± 8.7%. In contrast, no significant differences in \(\dot{\mathrm V}\)E and respiratory gas exchange ratio between the normoxia and hypoxia. The HR in hypoxia was marginally lower compared to normoxia (P < 0.1).
Cardiorespiratory variables across the four O2 levels are shown in Table 2. \(\dot{\mathrm V}\)E and HR progressively increased, while SaO2 progressively decreased. Below the 15% O2 level, there were significant differences in \(\dot{\mathrm V}\)E, HR, and SaO2 compared with those at 21% and 18% O2 (all P < 0.05, Table 2). Moreover, HR and SaO2 at 12% O2 were significantly higher and lower, respectively, compared with those at 15% O2 (P < 0.05). ΔSaO2 progressively increased from 18 to 12% O2, with significant differences between each oxygen level (all P < 0.05). HVR significantly increased from 18 to 15% O2, after which the value stabilized. No significant differences were observed in the HCR across the four O2 levels (all P > 0.05, Table 3). Figure 3 shows the relationships between oxygen level-induced changes in \(\dot{\mathrm V}\)O2peak and in SaO2, HVR, and HCR. Changes in \(\dot{\mathrm V}\)O2peak were associated with changes in SaO2 from 21 to 18 and 15% O2 and in the HCR from 21 to 12% O2 (Fig. 3A, B, and I, P < 0.05), while other cardiorespiratory variables were not associated with changes in \(\dot{\mathrm V}\)O2peak (Fig. 3C–H, all P > 0.05). Relative changes in \(\dot{\mathrm V}\)O2peak were explained by the following equation using multiple regression analysis:
When using partial correlation coefficients, the contribution rate of each variable was 70.6% of HCR and 29.4% of SaO2 for changes in \(\dot{\mathrm V}\)O2peak.
Discussion
We found that HCR and arterial desaturation, rather than HVR, may be associated with changes in \(\dot{\mathrm V}\)O2peak. Arterial desaturation increased with decreasing fractions of inspired O2. \(\dot{\mathrm V}\)E and HR also increased, possibly due to stimulation of peripheral chemoreceptors [21], increased sympathetic activity, and vagal withdrawal [22].
HVR reportedly significantly increased with decreasing O2 levels (14.5–12.7–11.5% O2) [7], which was partly inconsistent with our findings. Additionally, we found no significant relationships between ΔHVR and Δ\(\dot{\mathrm V}\)O2peak at all O2 levels (Fig. 3D–F), which contradicted a previous study [5]. These discrepancies may be related to HVR assessments across different time domains. In this study, the HVR at 12% O2 was assessed after 2 h 25 min exposure to hypoxia (Fig. 1), whereas a previous study assessed the HVR during the first 4 min of hypoxic exposure [7]. An initial rise in \(\dot{\mathrm V}\)E was followed by a marked decline after several minutes to an intermediate value over 25 min of hypoxia [15, 23]. Thus, our longer-term measurements may cause underestimation of increases in \(\dot{\mathrm V}\)E and HVR compared to previous studies [5, 7]. However, we found the HVR at 15% O2 was significantly greater than at 18% O2, suggesting light hypoxia at 18% O2 is not a sufficient stimulus to \(\dot{\mathrm V}\)E responses compared with 15% O2 (Table 2).
It should be noted that greater individual variance of HCR, calculated by dividing ΔHR by ΔSaO2, was observed in the present study (Table 2 and Fig. 3). In hypoxia, previous studies demonstrated that changes in SaO2 were inversely associated with ratio of low-frequency to high-frequency (frequency domain of heart rate variability) [24] and proportionally associated with root mean square successive difference (time domain of heart rate variability) [25]. Thus, changes in HCR can be partly contributed to a balance of cardiac autonomic nervous activity. Importantly, interindividual variations of heart rate variability has been considerably greater [26], which might explain greater individual variance of HCR in the present study.
Another possible explanation may be arterial baroreflex function rather than pulmonary inflation of reflex, which is caused by vagal withdrawal to hypoxic exposure [27]. However, we must acknowledge that this hypothesis is speculative, and therefore, future studies should be warranted.
The ΔSaO2 in this study was greater than that found in a previous study [7] (i.e., 7.6% at 15% O2 and 16.9% at 12% O2 vs. 4.3% at 14.5% O2 and 9.9% at 11.7% O2). Additionally, unlike a previous study [7], we found no significant differences in the HCR across different hypoxia levels. As greater ΔSaO2 values lead to lower HCR, a greater reduction in SaO2 may have mitigated the increase in HCR despite the elevation of HR. Although the detailed physiological mechanisms of ΔSaO2 in hypoxia are uncertain, population features might be related with these inconsistencies. Specifically, the participants in the previous study had no history of acute mountain sickness, whereas about 60% of our participants experienced symptoms of AMS [9]. The degree of oxygen desaturation has been reported as an important predictor of acute mountain sickness [8, 16, 28,29,30,31].
Notably, we found significant relationships between Δ\(\dot{\mathrm V}\)O2peak and hypoxic-induced cardiac responses (Fig. 3A, B, and I). Exercise-induced arterial hypoxemia influences the decline of \(\dot{\mathrm V}\)O2max in mild hypoxia [32, 33]. Additionally, greater arterial desaturation and lower \(\dot{\mathrm V}\)O2peak were reportedly observed in chronic obstructive pulmonary disease patients compared with those in healthy populations [34], suggesting a relation between arterial desaturation and aerobic capacity in healthy and diseases populations. The absence of a correlation between ΔSaO2 and Δ\(\dot{\mathrm V}\)O2peak at 21–12% O2 in this study suggests elevated HR can compensate arterial desaturation (Fig. 3I).
To clarify the contributions of each index on the decline in \(\dot{\mathrm V}\)O2peak, the optimal model could reveal further insight. The model showed that the contribution rate of HCR to changes in \(\dot{\mathrm V}\)O2peak is approximately 2.3 times than that of SaO2. This suggests that HR responses to greater arterial desaturation under severe hypoxia at rest play an important role in preventing declines in \(\dot{\mathrm V}\)O2peak at moderate hypoxia. One study exhibited more than double circulatory (HR) than respiratory (\(\dot{\mathrm V}\)E) energy expenditure costs in hypoxia regardless of rest or exercise [35], which could support these findings.
Study limitations and strengths
A major strength of this study is the relatively simple method using a multistep resting hypoxia test to assess the hypoxic exercise-induced declines in peak aerobic capacity. However, several limitations include small sample size, limited physiological variables as other possible predictors, and the retrospective analysis of data [9]. Second, we recruited only young men for the present study. Previous studies reported that sex- and age-related hypoxic ventilatory and/or cardiac responses were different between men and women [36, 37] or between young and old adults [36]. Thus, to generalize our results for wider population, future studies are warranted including women and/or aged people. Finally, during an incremental exercise test, we could not measure SaO2 that has been advocated as one potential candidate to decrease in \(\dot{\mathrm V}\)O2peak in a previous study [32].
In conclusion, this study suggests that arterial desaturation at moderate hypoxia and cardiac responses at severe hypoxia may account for hypoxic-induced declines in peak aerobic capacity, and hypoxic ventilatory responses may be unrelated, at least, in the population of the present study.
Availability of data and materials
The data of this study are available from the corresponding author upon reasonable request.
Abbreviations
- ANOVA:
-
Analysis of variance
- FiO2 :
-
Fraction of inspired oxygen
- HCR:
-
Hypoxic cardiac response
- HR:
-
Heart rate
- HVR:
-
Hypoxic ventilatory response
- SaO2 :
-
Peripheral arterial oxygen saturation
- SD:
-
Standard deviation
- \(\dot{\mathrm V}\) E :
-
Pulmonary ventilation
- VIF:
-
Variance inflation factor
- \(\dot{\mathrm V}\)O2peak :
-
Peak oxygen uptake
References
Fulco CS, Rock PB, Cymerman A. Maximal and submaximal exercise performance at altitude. Aviat Space Environ Med. 1998;69:793–801.
DeLorey DS, Shaw CN, Shoemaker JK, Kowalchuk JM, Paterson DH. The effect of hypoxia on pulmonary O2 uptake, leg blood flow and muscle deoxygenation during single-leg knee-extension exercise. Exp Physiol. 2004;89:293–302.
Horiuchi M, Handa-Kirihara Y, Abe D, Fukuoka Y. Combined effects of exposure to hypoxia and cool on walking economy and muscle oxygenation profiles at tibialis anterior. J Sports Sci. 2019;37:1638–47.
Byrne-Quinn E, Weil JV, Sodal IE, Filley GF, Grover RF. Ventilatory control in the athlete. J Appl Physiol. 1971;30:91–8.
Ogawa T, Hayashi K, Ichinose M, Nishiyasu T. Relationship between resting ventilatory chemosensitivity and maximal oxygen uptake in moderate hypobaric hypoxia. J Appl Physiol. 2007;103:1221–6.
Sheel AW, Koehle MS, Guenette JA, Foster GE, Sporer BC, Diep TT, et al. Human ventilatory responsiveness to hypoxia is unrelated to maximal aerobic capacity. J Appl Physiol. 2006;100:1204–9.
Lhuissier FJ, Brumm M, Ramier D, Richalet JP. Ventilatory and cardiac responses to hypoxia at submaximal exercise are independent of altitude and exercise intensity. J Appl Physiol. 2012;112:566–70.
Karinen HM, Peltonen JE, Kähönen M, Tikkanen HO. Prediction of acute mountain sickness by monitoring arterial oxygen saturation during ascent. High Alt Med Biol. 2010;11(4):325–32.
Horiuchi M, Endo J, Dobashi S, Kiuchi M, Koyama K, Subudhi AW. Effect of progressive normobaric hypoxia on dynamic cerebral autoregulation. Exp Physiol. 2016;101:1276–84.
Foster GE, McKenzie DC, Milsom WK, Sheel AW. Effects of two protocols of intermittent hypoxia on human ventilatory, cardiovascular and cerebral responses to hypoxia. J Physiol. 2005;567:689–99.
Chapman RF, Stray-Gundersen J, Levine BD. Individual variation in response to altitude training. J Appl Physiol. 1998;85(4):1448–56.
Roach RC. Cardiovascular regulation during hypoxia. In: Saltin B, Boushel R, Secher N, Mitchell H, editors. Exercise and circulation in health and disease. USA: Human Kinetics; 2000. p. 177–94.
Wilber RL. Application of altitude/hypoxic training by elite athletes. Med Sci Sports Exerc. 2007;39:1610–24.
Dobashi S, Horiuchi M, Endo J, Kiuchi M, Koyama K. Cognitive function and cerebral oxygenation during prolonged exercise under hypoxia in healthy young males. High Alt Med Biol. 2016;17:214–21.
Easton PA, Slykerman LJ, Anthonisen NR. Ventilatory response to sustained hypoxia in normal adults. J Appl Physiol. 1986;61:906–11.
Richalet JP, Larmignat P, Poitrine E, Letournel M, Canoui-Poitrine F. Physiological risk factors for severe high-altitude illness: a prospective cohort study. Am J Respir Crit Care Med. 2012;185:192–8.
Lakens D. Calculating and reporting effect sizes to facilitate cumulative science: a practical primer for t-tests and ANOVAs. Front Psychol. 2013;4:863. https://doi.org/10.3389/fpsyg.2013.00863.
Zuur AF, Ieno EN, Smith GM. Monitoring for change: Using generalized least squares, non-metric multidimensional scaling, and Mantel test on western Montana grasslands. In: Zuur AF, Ieno EN, Smith GM, editors. Analysing Ecological Data. New York: Springer; 2007. p. 463–84.
Horiuchi M, Watanabe M, Mitsui S, Uno T. Does change in barometric pressure per given time at high altitude influence symptoms of acute mountain sickness on Mount Fuji? A pilot study. J Physiol Anthropol. 2021;40(1):6. https://doi.org/10.1186/s40101-021-00256-y.
Uno T, Fujino M, Ohwaki A, Horiuchi M. Prevalence of falls on Mount Fuji and associated with risk factors: a questionnaire survey study. Int J Environ Res Public Health. 2019;16(21):4234. https://doi.org/10.3390/ijerph16214234.
Heistad DD, Abboud FM, Dickinson W. Richards lecture: circulatory adjustments to hypoxia. Circulation. 1980;61:463–70.
Koller EA, Drechsel S, Hess T, Macherel P, Boutellier U. Effects of atropine and propranolol on the respiratory, circulatory, and ECG responses to high altitude in man. Eur J Appl Physiol Occup Physiol. 1988;57:163–72.
Morrill CG, Meyer JR, Weil JV. Hypoxic ventilatory depression in dogs. J Appl Physiol. 1975;38:143–6.
Krejčí J, Botek M, McKune AJ. Dynamics of the heart rate variability and oxygen saturation response to acute normobaric hypoxia within the first 10 min of exposure. Clin Physiol Funct Imaging. 2018;38(1):56–62.
Botek M, Krejčí J, De Smet S, Gába A, McKune AJ. Heart rate variability and arterial oxygen saturation response during extreme normobaric hypoxia. Auton Neurosci. 2015;190:40–5.
Kobayashi H. Inter- and intra-individual variations of heart rate variability in Japanese males. J Physiol Anthropol. 2007;26(2):173–7.
Siebenmann C, Ryrsø CK, Oberholzer L, Fisher JP, Hilsted LM, Rasmussen P, et al. Hypoxia-induced vagal withdrawal is independent of the hypoxic ventilatory response in men. J Appl Physiol. 2019;126(1):124–31.
Burtscher M, Flatz M, Faulhaber M. Prediction of susceptibility to acute mountain sickness by SaO2 values during short-term exposure to hypoxia. High Alt Med Biol. 2004;5:335–40.
Burtscher M, Philadelphy M, Gatterer H, Burtscher J, Faulhaber M, Nachbauer W, et al. Physiological responses in humans acutely exposed to high altitude (3480 m): minute ventilation and oxygenation are predictive for the development of acute mountain sickness. High Alt Med Biol. 2019;20:192–7.
Faulhaber M, Wille M, Gatterer H, Heinrich D, Burtscher M. Resting arterial oxygen saturation and breathing frequency as predictors for acute mountain sickness development: a prospective cohort study. Sleep Breath. 2014;18:669–74.
Roach RC, Greene ER, Schoene RB, Hackett PH. Arterial oxygen saturation for prediction of acute mountain sickness. Aviat Space Environ Med. 1998;69:1182–5.
Chapman RF, Emery M, Stager JM. Degree of arterial desaturation in normoxia influences VO2max decline in mild hypoxia. Med Sci Sports Exerc. 1999;31:658–63.
Gaston AF, Durand F, Roca E, Doucende G, Hapkova I, Subirats E. Exercise-induced hypoxaemia developed at sea-level influences responses to exercise at moderate altitude. PLoS One. 2016;11(9):e0161819. https://doi.org/10.1371/journal.pone.0161819.
Gonzalez-Garcia M, Barrero M, Maldonado D. Exercise Capacity, Ventilatory response, and gas exchange in COPD patients with mild to severe obstruction residing at high altitude. Front Physiol. 2021;12:668144. https://doi.org/10.3389/fphys.2021.668144.
Horiuchi M, Fukuoka Y, Handa Y, Abe D, Pontzer H. Measuring the energy of ventilation and circulation during human walking using induced hypoxia. Sci Rep. 2017;7(1):4938. https://doi.org/10.1038/s41598-017-05068-8.
Camacho-Cardenosa A, Camacho-Cardenosa M, Tomas-Carus P, Timón R, Olcina G, Burtscher M. Acute physiological response to a normobaric hypoxic exposure: sex differences. Int J Biometeorol. 2022;66(7):1495–504.
Richalet JP, Lhuissier F, Jean D. Ventilatory response to hypoxia and tolerance to high altitude in women: influence of menstrual cycle, oral contraception, and menopause. High Alt Med Biol. 2020;21(1):12–9.
Acknowledgements
We appreciate the time and effort spent by our volunteer participants. We would like to thank Miss. Junko Endo for her technical assistant. The authors would like to thank Editage (https://www.editage.jp) for the English language service
Funding
This work was supported in part by Japan Society for the Promotion of Science (JSPS, KAKENHI, Number; 26440268 to M.H., and 17K01757 to K.K).
Author information
Authors and Affiliations
Contributions
The M.H., K.K., and Y.F. conceived and designed the study. M.H., S.D., and M.K. performed the experiments. M.H., S.D., M.K., and Y.F. analyzed data. M.H., Y.F., and K.K. interpreted results. M.H. prepared tables and figures. M.H. drafted the first manuscript. S.D., M.K., Y.F., and K.K. critically revised the manuscript. All authors approved the final version of the manuscript.
Corresponding author
Ethics declarations
Ethics approval and consent to participate
Following the Declaration of Helsinki, all participants provided written informed consent after being provided with information about the purposes, experimental protocols, and possible risks of this study. The ethical committee established in Mount Fuji Research Institute approved all procedures.
Competing interests
The authors declare that they have no competing interests.
Additional information
Publisher’s Note
Springer Nature remains neutral with regard to jurisdictional claims in published maps and institutional affiliations.
Rights and permissions
Open Access This article is licensed under a Creative Commons Attribution 4.0 International License, which permits use, sharing, adaptation, distribution and reproduction in any medium or format, as long as you give appropriate credit to the original author(s) and the source, provide a link to the Creative Commons licence, and indicate if changes were made. The images or other third party material in this article are included in the article's Creative Commons licence, unless indicated otherwise in a credit line to the material. If material is not included in the article's Creative Commons licence and your intended use is not permitted by statutory regulation or exceeds the permitted use, you will need to obtain permission directly from the copyright holder. To view a copy of this licence, visit http://creativecommons.org/licenses/by/4.0/. The Creative Commons Public Domain Dedication waiver (http://creativecommons.org/publicdomain/zero/1.0/) applies to the data made available in this article, unless otherwise stated in a credit line to the data.
About this article
Cite this article
Horiuchi, M., Dobashi, S., Kiuchi, M. et al. Hypoxic-induced resting ventilatory and circulatory responses under multistep hypoxia is related to decline in peak aerobic capacity in hypoxia. J Physiol Anthropol 41, 36 (2022). https://doi.org/10.1186/s40101-022-00310-3
Received:
Accepted:
Published:
DOI: https://doi.org/10.1186/s40101-022-00310-3