Abstract
Background
Heat stress is a major abiotic stress affecting the growth and development of plants, including crop species. Plants have evolved various adaptive strategies to help them survive heat stress, including maintaining membrane stability, encoding heat shock proteins (HSPs) and ROS-scavenging enzymes, and inducing molecular chaperone signaling. Brassinosteroids (BRs) are phytohormones that regulate various aspects of plant development, which have been implicated also in plant responses to heat stress, and resistance to heat in Arabidopsis thaliana is enhanced by adding exogenous BR. Brassinazole resistant 1 (BZR1), a transcription factor and positive regulator of BR signal, controls plant growth and development by directly regulating downstream target genes. However, the molecular mechanism at the basis of BR-mediated heat stress response is poorly understood. Here, we report the identification of a new factor critical for BR-regulated heat stress tolerance.
Results
We identified ERF49 in a genetic screen for proteins required for BR-regulated gene expression. We found that ERF49 is the direct target gene of BZR1 and that overexpressing ERF49 enhanced sensitivity of transgenic plants to heat stress. The transcription levels of heat shock factor HSFA2, heat stress-inducible gene DREB2A, and three heat shock protein (HSP) were significantly reduced under heat stress in ERF49-overexpressed transgenic plants. Transcriptional activity analysis in protoplast revealed that BZR1 inhibits ERF49 expression by binding to the promoter of ERF49. Our genetic analysis showed that dominant gain-of-function brassinazole resistant 1-1D mutant (bzr1-1D) exhibited lower sensitivity to heat stress compared with wild-type. Expressing ERF49-SRDX (a dominant repressor reporter of ERF49) in bzr1-1D significantly decreased the sensitivity of ERF49-SRDX/bzr1-1D transgenic plants to heat stress compared to bzr1-1D.
Conclusions
Our data provide clear evidence that BR increases thermotolerance of plants by repressing the expression of ERF49 through BZR1, and this process is dependent on the expression of downstream heat stress-inducible genes. Taken together, our work reveals a novel molecular mechanism mediating plant response to high temperature stress.
Similar content being viewed by others
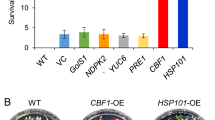
Background
As sessile and autotrophic organisms, terrestrial plants are constantly challenged by unsuitable environmental changes. Plants respond and adapt to environmental changes primarily by regulating external morphology and internal physiological characteristics. High temperature stress during plant growth and development processes caused by global warming is a critical issue in agriculture and ecology. Heat stress leads to severely reduced crop yield and degraded quality [1]. In plants, high temperatures induce changes in morphology, anatomy, and biochemistry at all levels of organization. At very high temperatures (more than 40 °C for Arabidopsis), severe cellular injury can occur and rapidly cause cell death. At moderately high temperatures (around 28 to 37 °C), obvious deleterious effects in plant growth and reproduction occur over a relatively long time [2]. Injuries caused by high temperature are mainly due to the denatured and aggregated proteins, increased membrane lipid fluidity, inactivated enzymes, altered microtubule organization, and disrupted cytoskeleton structures [3, 4]. Heat stress may reduce ion flux and increase production of reactive oxygen species (ROS) and other toxic compounds, which severely affect plant growth [3, 5].
To cope with heat stress, plants have evolved various mechanisms, including maintaining membrane stability, encoding heat shock proteins (HSPs) and ROS-scavenging enzymes, and inducing molecular chaperone signaling [6]. In higher plants, HSPs are extremely rapidly and intensively induced in a wide variety of cells and organisms to trigger thermotolerance. Five classes of HSPs have been distinguished by molecular weight: HSP100, HSP90, HSP70, HSP60, and small HSPs (15–30 kDa) [7, 8]. Many studies assert that the contribution of HSPs to heat tolerance is to act as molecular chaperones to maintain and/or restore the homeostasis of cell proteins under heat stress. For instance, HSP70 and HSP90 facilitate a variety of important processes including protein folding, protein transport, oligomeric protein assembly, and reactivation of denatured proteins [9, 10]. Moreover, heat shock factors (HSFs) are the key components mediating the activation of genes responsive to heat stress. They recognize and bind heat shock elements (HSEs), which are palindromic binding motifs located in the promoters of heat shock genes, conferring plant thermotolerance [11]. The 21 HSFs encoded in the A. thaliana genome can be categorized into three major classes (HSFA, HSFB, HSFC), based on the peculiarities of their oligomerization domain [12].
Brassinosteroids (BRs), polyhydroxylated steroidal phytohormones, regulate diverse aspects of plant biology, including elongation and division of cells, root development, stomatal and vascular differentiation, and seed germination [13, 14]. BRs also mediate plant responses to stress, including drought, salinity, disease, heat, cold, and nutrient deficiency [15, 16]. Numerous components of BR signaling have been identified in plants [17, 18]. BZR1 (Brassinazole resistant 1) is a positive regulator of BR signaling with dual roles in activating BR responses and regulating negative feedback of BR biosynthesis [19]. BZR1 activates BR responses by modulating its direct target genes [20, 21]. According to a previous study, control of thermomorphogenesis by phytochrome interacting factor 4 (PIF4) is dependent on BZR1 activity [22]. They found that BZR1 is a true, temperature-dependent positive regulator of PIF4, acting as a major growth coordinator. However, the molecular mechanisms of BZR1 and BR signaling pathways that mediate plant response to high temperature stress are still unclear.
The ERF (ethylene responsive factors) family is one of the largest families of transcription factors involved in a variety of biological processes related to growth and development, as well as various responses to environmental stimuli [23]. ERFs, belonging to the AP2/ERF multi-gene family, are known to be the integrative node in ethylene signaling and in the regulation of several stress-responsive genes and also common transcription factors of different signaling pathways in Arabidopsis [24]. Ectopic expression of a number of ERF genes enhances the tolerance of plants to heat stress, indicating that ERFs participate in heat stress response [25, 26]. However, the role of ERF-mediated heat stress response remains unclear. In this study, we identified ERF49 in a genetic screen for proteins critical to BR-regulated gene expression. ERF49 is one member of AP2/ERF family which functions directly in diverse plant developmental and physiological processes. We showed that ERF49 promoter physically interacts with BZR1, which inhibits the expression of ERF49. Dominant inhibition of ERF49 enhanced the thermotolerance of plants by increasing the expression of downstream heat stress-inducible genes under high temperature, and ERF49 participated in the plant response to high temperature mainly through the BZR1 pathway. Together, our results identified an important transcription factor in process of how BR-enhanced plants cope with heat stress, laying a foundation for in-depth studies on the molecular mechanism of BR-enhanced thermotolerance in plants and further molecular improvement of crop tolerance to high temperature conditions.
Results
BR enhanced heat stress tolerance in Arabidopsis thaliana
One study has shown that applying exogenous 2, 4- epi-brassinosteroid (eBL) increases the heat resistance of rape and tomato seedlings [27] and reported that BR increased the basal heat resistance of A. thaliana seedlings [28]. To verify these results, we first examined the effect of exogenous application of 2, 4-eBL on the response of A. thaliana Col-0 to high temperature. Compared with the control group, the survival rate of A. thaliana treated with 10 nM 2, 4-eBL increased significantly under high temperature stress (54.4 %), while the survival rate of A. thaliana treated with 100 nM 2, 4-eBL was the highest (92.2 %) (Fig. 1a, b).
Exogenous 2, 4-eBL treatment and endogenous BR increase thermotolerance of Arabidopsis thaliana. a The influence of different concentrations of 2, 4-eBL on heat tolerance of Arabidopsis thaliana. b Survival rate of A. thaliana treated with 2, 4-eBL in a heat stress environment. c The thermotolerance of BR biosynthesis and signaling transduction mutants. d The survival rate of BR biosynthesis and signaling transduction mutants after heat stress treatment. Seven-day-old seedlings were treated under 42 °C for 3 h and recovery for 5 days. Sixty to eighty seedlings of each genotype from three biological replicates were counted. Asterisks represent statistical significance (****, P < 0.0001; one-way ANOVA followed by post hoc Tukey’s multiple comparison test). Numerical data is provided in Additional file 6. Experiments were repeated for three times, the error bars indicate standard deviation
To further study the effect of endogenous BR on plant response to high temperature, we examined the response of BR-related mutants in A. thaliana to high temperature. As shown in Fig. 1c and d, det2 (in the Arabidopsis ecotype Columbia (Col-0) background) and bri1-5 (in the Arabidopsis ecotype Wassilewskija (WS) background) plants were more sensitive to high temperature compared with wild-type plants. det2 and bri1-5 had lower survival rates under high temperature stress (respectively 23 %, 60 %) than wild-type plants (35 %, 96 %), while the dominant gain-of-function mutant bzr1-1D was less sensitive to high temperature and had higher survival rates (79 %) than wild-type Col-0 (35 %) under high temperature stress. Together, the results indicate that BR enhances the tolerance of plants to high temperature and that overexpression of BZR1 can enhance BR-induced heat stress tolerance.
ERF49 structure prediction and characterization
Based on the chromatin immunoprecipitation microarray (ChIP-chip) analysis of BZR1, it was found that almost 1/3 of the members of AP2/ERF family are BR-regulated BZR1 target (BRBT) genes [21]. Many studies showed that the AP2/ERF transcription factor family is closely related to plant response to stress [29,30,31]. Further expression analysis of BZR1 and target genes in AP2/ERF family by Arabidopsis eFP Browser showed that the absolute expression of ERF49 and BZR1 is consistently similar in most tissues (Additional file 1: Fig. S1), revealing that ERF49 may be the target gene of BZR1 and play the role in plant resistance to stress.
ERF49 protein belongs to group IV according to analysis of the phylogeny and conserved domains of the AP2/ERF family in Arabidopsis and is one member of the DREB subfamily of the AP2/ERF family [23]. Members of DREB subfamily specifically bind to the dehydration-responsive element (DRE)/C-repeat (CRT) cis-acting element (A-GCCGAC) and control the gene expression responsive to drought and low-temperature stress in Arabidopsis [32]. ERF49 is 206 amino acids long and has a molecular weight of 22.6 kDa. As predicted, it contains a single DNA-binding domain (the AP2/ERF domain) and the motif CMIV-1 (Fig. 2a). CMIV-1 is 27 amino acid residues defined by the conserved amino acid motif [K/R] GKGGPxN and predicted to contain a nuclear localization sequence (NLS) [33].
The structure and characterization of ERF49. a The gene and protein structure of ERF49. The exon is denoted by a black box. 5′-UTR and 3′-UTR are denoted by white boxes. The translation initiation codons are shown. aa means amino acids and bp means base pair. b GUS staining assay of ERF49pro::gERF49-GUS transgenic plants in different tissues (from left to right: 14-day-old seedling, rosette leaf (upper), 5-day-old seedling (upper), silique (lower), inflorescence (upper), and cauline leaf (lower)). Scale bars, 5 mm. c Analysis of ERF49 expression level in different organs by quantitative reverse transcription-polymerase chain reaction (RT-qPCR). The expression level in the seedlings is set to 1.0, and error bars represent standard deviations of three biological replicates. Numerical data is provided in Additional file 6. d Confocal micrographs of subcellular localization of reporter signal exhibited by 35S::ERF49-GFP in tobacco leaf. Scale bars: 50 μm
To further investigate the characterization of ERF49 in A. thaliana, we generated transgenic Arabidopsis seedlings expressing a ERF49pro::gERF49-GUS transgene. The results of GUS staining showed that ERF49 protein was mainly accumulated in seedlings, hypocotyl, and rosette leaves and weakly accumulated in stems, inflorescences, silique, and sepals (Fig. 2b). The expression analysis using quantitative reverse transcription PCR (RT-qPCR) showed that ERF49 was highly expressed in seedlings, siliques, leaf, and flower (Fig. 2c). Furthermore, ERF49-GFP (green fluorescent protein) fusion protein transiently expressed from the constitutive 35S promoter (35S::ERF49-GFP) in tobacco leaf cells showed that fluorescent signals were mainly in nuclei (Fig. 2d).
ERF49 is a negative regulator in plant thermotolerance
Our RT-qPCR analysis revealed that the expression of ERF49 was decreased at the transcriptional level after high temperature treatment (Fig. 3a), indicating that ERF49 might be a negative regulator in heat stress responses. In order to test this speculation, we generated transgenic plants overexpressing ERF49 (ERF49-OX) or ERF49 fused to the SRDX transcriptional repression domain driven by the CaMV35S promoter (ERF49-SRDX) in the Col-0 background. The SRDX motif is the modified version of the EAR-motif repression domain to generate a chimeric repressor from the transcription factors. This dominant repressor also represses the activity of the functionally redundant homologs [34, 35]. The relative expression levels of ERF49 transgenic plants under Col-0 are shown in Fig. 3b and c. The relative expression of ERF49 in overexpressing lines OX-3, OX-11, and OX-39 was significantly increased compared with Col-0 (Fig. 3b). ERF49-SRDX expression was also significantly increased in SRDX-14, SRDX-17, and SRDX-19, indicating that it significantly suppressed the activity of ERF49 (Fig. 3c). Analysis of the survival rate showed that the overexpressed transgenic plants decreased in size and survival rate (mean about 25 %), compared with wild-type (mean about 52 %) after high temperature treatment. The dominant inhibited transgenic plants were larger in overall size than the wild-type plants, and their survival rate (mean about 73 %) was significantly higher than that of the wild-type plants (Fig. 3d, e).
ERF49 affects heat stress responses in Arabidopsis thaliana. a The relative expression level of ERF49 in Arabidopsis seedlings after heat treatment for 0, 30, 60, 90, and 120 min in a 42°C environment. b The relative expression level of ERF49 in three ERF49-overexpression transgenic lines. c The relative expression level of ERF49 in three ERF49-dominant-negative transgenic lines. d The phenotype of ERF49-overexpression and dominant-negative transgenic lines after heat stress treatment at 42°C for 0 h (top panel) and 3 h and recovery for 5 days (middle and bottom panels). The bottom panel shows the phenotype of 3 random seedlings in each ERF49 transgenic line in the middle panel graph. Col-0 is control. e The survival rates of ERF49 overexpression and dominant-negative transgenic lines after heat stress treatment at 42°C for 3 h and recovery for 5 days; 60–80 seedlings of each genotype from three biological replicates were counted. Col-0 is control. Asterisks represent statistical significance (*, P < 0.05; **, P < 0.01; ***, P < 0.001; ****, P < 0.0001; one-way ANOVA followed by post hoc Tukey’s multiple comparison test). UBC30 was used as a reference gene. Numerical data is provided in Additional file 6. The experiments were repeated three times, error bars indicate standard deviation
Considering that the induction of HSPs is a central component of responses to heat stress in plants, we determined the expression levels of several representative HSPs in transgenic plants (Additional file 1: Fig. S2a-c). The expression of HSP26.5, HSP70, and HSP90.1 were slightly different in transgenic plants under control conditions. Differently, the transcript levels of these HSPs in ERF49-OX plants were significantly reduced compared with the wild-type under heat stress. In contrast, transcript levels of these HSPs were significantly higher in ERF49-SRDX plants than in wild-type plants under heat stress. Since heat induces HSPs mainly via HSFs, we also examined whether ERF49 regulates HSFs expression. The results showed that the expression of HSFA2 and heat stress-inducible gene DREB2A in ERF49-OX plants were significantly reduced, but were significantly induced in ERF49-SRDX plants under heat stress (Additional file 1: Fig. S2d, e). Taken together, we speculated that ERF49 negatively regulates the tolerance of plants to high temperature by reducing the expression of downstream heat stress-inducible genes.
ERF49 is a direct target gene of BZR1, and its expression is repressed by BR and BZR1
ERF49 has been identified as BR-regulated BZR1 target gene by transcript profiling and ChIP-chip analysis [21] (Additional file 1: Fig. S3a). We further analyzed the promoter sequence of ERF49 and found that there are 12 putative BZR1 binding sites in ERF49 promoter: one BRRE (CGTGT/CG) and 11 E-BOX (CANNTG) [21, 36, 37] (Additional file 1: Fig. S3b). To verify the regulatory effect of BZR1 on ERF49, we conducted the yeast one-hybrid assay with BZR1 as prey and ERF49 promoter which divided into three fragments according to the distribution of BZR1 binding sites as bait. The results of assay showed that lacZ reporter gene activity was strongly activated when BZR1 and the second promoter fragment (ERF49pro-2) were simultaneously transformed into the yeast cells (Fig. 4a), indicating that BZR1 binds to the −420 to −1102 bp region of ERF49 promoter. In ChIP-qPCR assays, the F1 fragment with BRRE motif of ERF49 promoter sequence was enriched after immunoprecipitation of CFP-tagged BZR1 (Fig. 4b). A transcriptional activity assay demonstrated that co-expression with BZR1 reduced the LUC reporter gene activity driven by P2 fragment with BRRE motif. However, the LUC reporter gene activity driven by fragment with mutated BRRE motif was comparable to that in control (Fig. 4c–e). Consistently, the yeast one-hybrid experiment also showed that BZR1 interacted with P2 fragment with BRRE motif. The interaction was abolished when BRRE motif in ERF49 promoter fragment was mutated (Fig. 4f). These results indicate the BZR1 specifically binds to BRRE motif of ERF49 promoter. RT-qPCR analysis showed that ERF49 transcription was significantly decreased in the BR signal-enhanced dominant mutant bzr1-1D, but significantly increased in the BR-deficient mutant det2 compared with Col-0 (Fig. 4g). This pattern is consistent with suppression of the transcript level of ERF49 in Col-0 after treatment with 100 nM 2, 4-eBL (Fig. 4h). We also found that ERF49 protein level decreased in ERF49pro::gERF49-GUS transgenic plants after BR treatment by GUS staining (Fig. 4i). Taken together, these results provide direct evidence for BZR1 binding to the promoter of ERF49 gene in vivo and show that ERF49 is repressed by BR through BZR1.
Expression of ERF49, a direct target gene of BZR1, is repressed by BZR1 and BR. a Yeast one-hybrid assays for binding of BZR1 to the ERF49 promoter. Diagram depicting the segmentation of ERF49 promoter. Black and red asterisks indicate the positions of putative E-box and BRRE motifs, respectively. The region from −1964 to −1 bp was named ERF49pro and the region from −1102 to −420 bp was named ERF49pro-2 (upper panel). The activity of β-galactosidase when BZR1 and ERF49pro-2 were transformed into the yeast cells together (lower panel). b ChIP-qPCR assays for direct binding of BZR1 to the ERF49 promoter. Relative enrichment of PP2A coding region was normalized as an internal control. DWF4 and CPD were identified as target genes of BZR1. ERF49-F1 indicates the sequence analyzed by ChIP-qPCR. c Dual-luciferase reporter assay of ERF49 in Arabidopsis protoplasts. Schematic diagram of effector (35S::BZR1) and reporter (ERF49pro::LUC) constructs used in the protoplast transcription system (upper panel). Expression activity assay of LUC reporter genes in Arabidopsis protoplasts transformed with the effector and reporter plasmids (lower panel). The plasmid pUC18-3HA was empty vector (EV). d Transcriptional activity assays of the interaction between BZR1 protein and ERF49 promoter fragments. Tobacco transient expression of the LUC reporter gene (under the control of the ERF49 promoter fragments with native or mutant BRRE motif) by the BZR1. e Quantification of the dual-luciferase assays of LUC expression in d. The expression of REN (Renilla luciferase) was used as the internal control. The LUC/REN ratio indicates the relative activity of the promoter. Asterisks represent statistical significance (**, P < 0.01; one-way ANOVA followed by post hoc Tukey’s multiple comparison test). f Yeast one-hybrid assay of the interaction between BZR1 protein and ERF49 promoter fragments. Systematic yeast one-hybrid assay showing the binding of BZR1 to the ERF49 promoter fragments with native or mutant BRRE motif. g The relative expression level of ERF49 in Col-0, bzr1-1D, and det2. h Relative expression level of ERF49 in Col-0 after 100 nM 2, 4-eBL for 0, 1, 2, 4, 8, and 12 h. i GUS activities of the ERF49pro::gERF49-GUS transgenic Arabidopsis plants after soaking in a solution of 100 nM 2, 4-eBL for 0 and 4 h. CK was a solution containing 80% ethanol. Scale bars, 2 mm. Asterisks represent statistical significance (**, P < 0.01; ***, P < 0.001; ****, P < 0.0001; one-way ANOVA followed by post hoc Tukey’s multiple comparison test). UBC30 was used as a reference gene. Numerical data is provided in Additional file 6. The experiments were repeated three times, error bars indicate standard deviation
ERF49 is a positive regulator in the BR pathway
In order to further understand the role of ERF49 in BR signal transduction pathway, we generated the transgenic plants driven by CaMV35S promoter that fused ERF49 into the SRDX transcriptional repression domain in the bzr1-1D background (ERF49-SRDX/bzr1-1D). The relative expression level of transgenic plants is shown in Additional file 1: Fig. S4a. Firstly, we observed the above-ground growth of ERF49-SRDX/bzr1-1D transgenic plants. As shown in Fig. 5a, ERF49-SRDX/bzr1-1D transgenic lines displayed significantly smaller, narrowed, and curled down leaves with shorter petioles compared to bzr1-1D. Moreover, compared with Col-0, the flowering time of bzr1-1D mutant was delayed by about 1 week, but overexpression of ERF49-SRDX in bzr1-1D significantly promoted the flowering time of transgenic plants, close to that of the wild-type Col-0. In addition, distinct from bzr1-1D, the transgenic plants also showed shorter and curved siliques (Fig. 5a).
ERF49 is a positive regulator in the BR pathway. a The phenotype of ERF49 dominant-negative transgenic lines in a bzr1-1D background. Scale bar for the siliques, 5 mm. The rest of scale bar, 2.5 cm. b Analysis of hypocotyl elongation in the ERF49 transgenic plants with 2, 4-eBL or 80% ethanol (as control) treatment. Scale bar, 1 cm. Hypocotyl lengths of over 30 seedlings were measured using ImageJ for each independent line. The standardized data is shown with mock as 100% (lower panel). c Relative expression level of downstream gene CPD regulated by BR in the ERF49 transgenic lines. d Relative expression level of BR-regulated downstream genes XTR6 and SAUR_AC1 in bzr1-1D, det2, and ERF49 dominant-negative transgenic lines. Asterisks represent statistical significance (*, P < 0.05; ***, P < 0.001; ****, P < 0.0001; one-way ANOVA followed by post hoc Tukey’s multiple comparison test). UBC30 was used as a reference gene. Numerical data is provided in Additional file 6. The experiments were repeated three times, error bars indicate standard deviation
Low-concentration BL promotes hypocotyl elongation, while high-concentration BL inhibits elongation [19, 38, 39]. Analysis of hypocotyl elongation in ERF49 transgenic plants showed that hypocotyls of ERF49-OX plants were shorter than those of Col-0 on medium containing 100 nM 2, 4-eBL (Fig. 5b and Additional file 1: Fig. S4b, c), indicating that overexpression of ERF49 increased sensitivity to BR. RT-qPCR analysis showed that the expression level of the BR-responsive gene CPD, reported to be downregulated by BZR1 [36], decreased in ERF49-OX plants compared with Col-0, but increased in ERF49-SRDX plants (Fig. 5c). Two downstream response genes of BR signal (SaurAC1 and XTR6), which have very low expression in det2, were significantly induced in bzr1-1D, and reduced in ERF49-SRDX/bzr1-1D transgenic plants but still significantly higher than det2 (Fig. 5d). Together, these results suggest that ERF49 partially mediates multiple developmental, physiological, and molecular phenotypes and functions as a positive regulator in the BR signaling pathway.
BZR1 is involved in BR-mediated plant thermotolerance through ERF49 in Arabidopsis thaliana
Since overexpression of BZR1 enhanced BR-induced plant thermotolerance (Fig. 1c, d) and ERF49 is the direct target gene of BZR1, we decided to investigate whether the response of ERF49 to heat stress was regulated by BZR1. Here, we examined the response of ERF49-SRDX/bzr1-1D transgenic plants to heat stress, the survival analysis showed that the survival rate of ERF49-SRDX/bzr1-1D transgenic plants (around 60 %) was significantly higher than bzr1-1D (around 40 %) after 3 h heat stress treatment (Additional file 1: Fig. S5).
Furthermore, we selected four ERF49-SRDX/Col transgenic lines (SRDX-9/Col, SRDX-15/Col, SRDX-17/Col, SRDX-18/Col) and four ERF49-SRDX/bzr1-1D transgenic lines (SRDX-1/bzr1-1D, SRDX-9/bzr1-1D, SRDX-11/bzr1-1D, SRDX-4/bzr1-1D) to explore whether their thermotolerance was significantly different from their own background after heat stress treatment. The expression level of ERF49-SRDX in these transgenic lines had basically the same fold change relative to its own background (Col or bzr1-1D), that is, the degree of inhibition of ERF49 expression had the same level in the transgenic lines as the background (Fig. 6a). The results of survival rate analysis after heat stress treatment showed that ERF49-SRDX/bzr1-1D was strongly increased compared with ERF49-SRDX/Col. However, the trend of survival rate change of ERF49-SRDX/Col relative to Col-0 was the same as that of ERF49-SRDX/bzr1-1D relative to bzr1-1D (Fig. 6b, c). Furthermore, we also examined the thermotolerance of ERF49 transgenic plants in the presence of 2, 4-eBL or BRZ (brassinazole, BR biosynthesis inhibitor). The statistical results showed that in the presence of 2, 4-eBL, the survival rate of plants generally increased after high temperature treatment. Exogenous application of 2, 4-eBL resulted in higher survival rate of ERF49-SRDX plants, while the survival rate of ERF49-OX plants was not significant different from wild-type (Additional file 1: Fig. S6a). Conversely, in the presence of BRZ, the survival rate of plants decreased at high temperature in general (Additional file 1: Fig. S6b). Compared with wild-type, the survival rate of overexpressed transgenic plants significantly decreased, while that of ERF49-SRDX transgenic plants significantly increased (Additional file 1: Fig. S6b). Taken together, these results suggest that ERF49 is involved in the responsive to high temperature through the BR pathway.
The response of dominant-negative ERF49 in Col-0 or bzr1-1D to heat stress. a The relative expression level of ERF49 in eight ERF49 dominant-negative transgenic lines. b, c The phenotype and survival rates of ERF49 dominant-negative transgenic lines in Col-0 or bzr1-1D after heat stress treatment at 42 °C for 3 hours and recovery for 5 days. Sixty to eighty seedlings of each genotype from three biological replicates were counted. Col-0 and bzr1-1D were used as controls. Asterisks represent statistical significance (**, P<0.01; ***, P < 0.001; ****, P < 0.0001; one-way ANOVA followed by post hoc Tukey’s multiple comparison test). Numerical data is provided in Additional file 6. The experiments were repeated three times, error bars indicate standard deviation
The HSPs are in downstream of BR-mediated ERF49 regulated heat stress response
We next performed RNA-seq analysis of ERF49-overexpression lines after heat stress treatment. The RNA-seq of ERF49-overexpression lines was analyzed with published transcriptome data of heat and BR treatment in wild-type to further investigate the downstream BR-mediated ERF49 in regulating heat stress response [40, 41]. There are 1251 genes downregulated in ERF49 overexpression lines after heat treatment (Additional file 2). The 4125 and 1375 genes were upregulated in wild-type after heat and BR treatment, respectively (Additional file 3 and Additional file 4). There are 30 overlap genes downregulated in ERF49-overexpression lines under heat treatment and upregulated in wild-type after heat and BR treatment (Fig. 7a and Additional file 5). Gene Ontology (GO) enrichment analysis of 30 overlap genes showed that the heat and temperature response terms are the most enriched terms (Fig. 7b). There are eight HSP genes in these two enriched terms and all of them are downregulated in ERF49 overexpression lines under heat treatment (Fig. 7c) and upregulated in wild-type after heat and BR treatment (Fig. 7d, e). These results further confirmed that BR-mediated ERF49 regulates heat stress response by negatively regulating expression of HSP genes.
The HSPs are in downstream of BR and ERF49 in regulating heat stress response. a Venn diagram showing the overlap genes downregulated in ERF49-overexpression lines under heat stress and upregulated in wild-type after heat and BR treatment. b GO analysis of 30 overlap genes downregulated in ERF49-overexpression lines under heat stress and upregulated in wild-type after heat and BR treatment. c–e Heatmap of HSP genes in response to heat or temperature stimulus in WT and ERF49-overexpression plants (c), heat treatment (d), and BR treatment (e), respectively
Based on the data collected above, we propose a working model of BR-mediated heat stress response mechanism in which BR promotes plant thermotolerance through BZR1 directly inhibiting the expression of ERF49. In addition, ERF49 indirectly represses the HSPs expression to regulate the heat stress tolerance pathway (Fig. 8).
A model for ERF49-mediated BR regulation of heat stress tolerance. As a transcriptional activator, ERF49 positively regulates the downstream targets of BR signal transduction to promote plant development. When plants exposed to heat stress, the expression of ERF49, a direct target gene of BZR1, was inhibited. Meanwhile, ERF49 may activate of downstream HSPs-associated suppressors (X) in BR signal transduction, which directly or indirectly inhibit the expression of HSPs under high temperature stress
Discussion
BR, an important plant hormone, is essential in plant responses to stress, such as high temperature, drought, salinity, and cold [15, 16]. One study reported that BR increases the basal heat resistance of A. thaliana seedlings [28], but the molecular mechanism of how BR mediates plant response to heat stress is still not clear. By analyzing thermotolerance of Col-0 treated with 2, 4-eBL and the response of BR-related mutants to high temperature, we found that BR enhances the tolerance of plants to high temperature in A. thaliana (Fig. 1).
The BES1 (BRI1-EMS-SUPPRESSOR 1) and BZR1 (BRASSINAZOLE RESISTANT 1) are two key transcription factors in BR signaling pathway which directly bind to thousands of BR-responsive gene promoters after dephosphorylation and control their transcription. When BRs are absent, the GSK3-like kinase BIN2 (BRASSINOSTEROID INSENSITIVE 2) phosphorylates BES1 and BZR1 proteins and inactivates these two factors [16]. Recent studies reported that the addition of BR increased the thermotolerance of Arabidopsis, and BRASSINOSTEROID INSENSITIVE 2 (BIN2) plays a critical role in the regulation of plant heat stress responses [42]. The BES1, as a transcription regulator, can be dephosphorylated and directly bind to heat shock element of heat stress transcription factors to regulate the expression of HSFs to respond to heat shock [43]. The BZR1 regulates growth and development of plants by regulating expression of downstream target genes [20, 21]. Several studies have found that BZR1 and PIF4 form a heterodimer to jointly regulate the expression of downstream target genes and participate in the response to high temperature [22]. Our study also revealed that BR contributes to thermotolerance of Arabidopsis and identified a new direct target gene of BZR1, ERF49, which regulates heat stress response via indirectly regulating the expression of heat stress-related proteins.
ERF49, also named DREB2D according to the phylogenetic relationship, is a member of the DREB2 subfamily of AP2/ERF domain transcription factors [23]. Arabidopsis DREB2 transcription factors have been reported to be involved in various abiotic stress processes [44, 45]. The DREB2A was rapidly and transiently induced under heat stress treatment at 37 °C, resulting in the expression of many transcription factors or molecular chaperones encoded by heat shock response genes [46]. And the expression of HSFA3 was directly regulated by DREB2A under heat stress [46, 47]. Others previously showed that DREB2C is involved in heat responses and functions as a transcriptional activator of HSFA3 during the heat stress response [48, 49]. DREB2C has also been reported to play a regulatory role in ABA and stress response [50]. However, the functions of DREB2 family transcription factors except DREB2A and DREB2C are still unclear. Here, our results suggested that DREB2D (ERF49) is also involved in heat response. We showed that ERF49-SRDX lines exhibit the phenotype of heat stress tolerance (Fig. 3), indicating that ERF49 plays a negative regulatory role in plant thermotolerance. Furthermore, overexpression of ERF49 reduced the induction of HSFA2 and DREB2A by heat stress, indicating that ERF49 may play a regulatory role in heat stress by regulating the expression of HSFA2. The HSFA2 is a heat-inducible gene, which was reported to drive the transcriptional memory after heat stress via forming heteromeric complexes with HSFA3 and additional HSFs [51]. Recent research also reported that the HSFA2 directly activates the H3K27me3 demethylase REF6, which in turn de-represses HSFA2 and transmit plant thermotolerance [52]. Further investigation is needed into the specific regulatory mechanisms between ERF49 and HSFA2.
ERF49 functions as a positive regulator in the BR signal transduction pathway, because the dominant inhibitory mutant of EFR49 suppresses the phenotype of bzr1-1D (Fig. 5a). Why is the positive regulator ERF49 repressed by BRs and BZR1 in bzr1-1D? One possibility is that it represents a feedback regulatory mechanism to maintain the balanced level of BRs or BR signaling at which BZR1 regulates plant development through ERF49. This result is similar to the role of myeloblastosis family transcription factor-like 2 (MYBL2) in the BR signal transduction pathway [53]. The ERFs are key regulators in various biotic or abiotic stress response and capable of binding to different cis-element for different stress response, and for pathogen resistance response, the ERFs were reported to bind to GCC box of downstream genes [54]. For salt, drought, and heat stress response, the ERFs activate target genes for stress response by binding to a specific cis-acting dehydration-responsive element (DRE) (CCGAC) [25]. Our results suggested that ERF49 participates in the plant response to high temperature mainly through the BZR1 pathway (Fig. 6). The downstream target of ERF49 and the regulatory mechanism of ERF49 and its target for heat stress response need to be further investigated in the future.
As the direct target gene of BZR1, the expression of ERF49 positively regulates the activity of the downstream targets after being repressed by BZR1 (Fig. 5d). However, we found that ERF49 negatively regulates the activity of HSPs under high temperature (Additional file 1: Fig. 2). Why the transcriptional activator ERF49 represses the expression of HSPs, and thus negatively regulates the response to heat stress in A. thaliana? We speculate that the repression of HSPs by ERF49 is not a direct effect. One possibility is that ERF49 indirectly represses the HSPs expression by positively regulating the downstream HSPs-associated suppressors of BR signaling transduction (Fig. 8). Taking all of our data together, we hypothesized that BZR1 repressed the activity of downstream HSPs-associated suppressors by directly repressing the expression of ERF49, thereby indirectly promoting the expression of HSPs under high temperature stress, thus conferring the heat stress resistance in A. thaliana (Fig. 8).
Conclusions
Our results provide an updated model for the thermotolerance of plants in A. thaliana. It is of great significance to further study the downstream target genes of ERF49 under high temperature stress. Moreover, other unknown pathways may also be involved in the response process of ERF49 to high temperature. Accordingly, it will be interesting to further explore the exact mechanism responsible for plant thermotolerance.
Methods
Plant materials and growth conditions
Arabidopsis thaliana ecotype Columbia (Col-0) was used in this study as a wild-type control. bzr1-1D and BZR1pro::BZR1-GFP are in the Col-0 ecotype background and both were described previously [19]. bri1-5 is in the Wassilewskijia (WS) ecotype background [55]. Arabidopsis seeds were sterilized with 75% ethanol plus 0.01 % Triton X-100 for 15 min, then rinsed with 95% ethanol, and dried in a hood. Sterilized seeds were then sown on half-strength Murashige and Skoog (1/2 MS) medium, and plates with seeds were maintained at 4 °C for 2 days to break dormancy prior to transfer to a culture room at 22 °C under dark/light cycles of 8/16 h for 1 week. Positive transgenic seedlings, screened by applying hygromycin B in the medium, were transferred and grown in a controlled culture room with the same dark/light cycle conditions. For plant treatment, 2, 4-eBL stock solution was diluted to the working concentration in 1/2 MS medium.
Hypocotyl assay and survival assay under heat stress
In this study, 2, 4-eBL was used in all BR treatments. To detect seedling response to BR (2, 4-eBL), seeds were spotted on 1/2 MS medium containing 10 nM, 100 nM, 1 μM, and 10 μM 2, 4-eBL, vernalized for 2 days, and vertically cultured on 1/2 MS medium for 7 days. Phenotype analysis of hypocotyls was described previously [19].
For the survival assay under heat stress, 7-day-old seedlings grown on 1/2 MS medium were transferred to 42 °C for 3 h in dark, and then transferred into the culture room at 22 °C under dark/light cycles of 8/16 h for 5 days. In the statistics of survival rate, we counted the number of fully etiolated seedlings, partially etiolated seedlings, and non-etiolated seedlings after high temperature treatment. Survival rate = (number of non-etiolated seedlings + 1/2 number of partially etiolated seedlings) / total number of seedlings.
Plasmid construction and generation of transgenic plants
To obtain the overexpression vector of ERF49 (ERF49-OX), a 621-bp genomic fragment containing full-length ERF49 open reading frame (ORF) was amplified by PCR and ligated into pENTRTM/SD/D-TOPO vector, and then ligated into pMDC32 by recombinant cloning [56]. To get the dominant repressor of ERF49 (ERF49-SRDX), the full-length cDNA sequence was amplified by PCR. Then the segment was ligated into p35S-SRDX vector between BamHI and SpeI sites. To get localized expression of ERF49, a 2582-bp (without terminator codon) genomic segment containing promoter and gene sequence was amplified by PCR and ligated into pENTRTM/SD/D-TOPO vector, and then ligated into pMDC163 to produce ERF49pro::gERF49-GUS [56]. To obtain the vector of 35S::ERF49-GFP, a 621-bp genomic fragment containing full-length ERF49 open reading frame (ORF) was amplified by PCR and ligated into pENTRTM/SD/D-TOPO vector, and then ligated into pMDC83 by recombinant cloning [56]. The constructs were transformed into the Agrobacterium tumefaciens strain GV3101 and then introduced into A. thaliana plants via floral dip method [57].
The ERF49 promoter fragment with mutant BRRE motif (CGTCTC to TTTTTT) was synthesized via a biological company (ShengGong, Shanghai, China). For the dual-luciferase reporter assay, a 1.6-kb fragment upstream of the ERF49 translational start code and the corresponding DNA fragments with intact or mutated BRRE cis-element variant were PCR amplified and inserted into the SalI-BamHI site of the pGreenII0800-LUC vector, generating ERF49pro::LUC. The BZR1 open reading frame was PCR amplified and inserted into the MfeI-KpnI site of pUC18-3HA to produce 35S::BZR1 for the analysis in Arabidopsis protoplasts and inserted into the pGreenII 62-SK vector for the analysis in tobacco to produce pGreenII 62-SK::BZR1.
Total RNA extraction and quantitative RT-qPCR analysis
Total RNA was extracted from Arabidopsis seedlings using Trizol reagent (Invitrogen, Carlsbad, California, USA). First-strand cDNA was synthesized with a reverse transcript kit (Vazyme, China) and used as RT-qPCR templates. Quantitative real-time PCR analyses were carried out on an ABI7500 (Applied Biosystems, Foster City, California, USA) using the SYBR@ Green reagent (Toyobo, Osaka, Japan) according to the manufacturer’s instructions. The RT-qPCR was repeated at least three times using samples harvested separately. The UBC30 gene was used as an internal reference. The primer sequences used for RT-qPCR are in Additional file 1: Table S1.
Assays of GUS activity
Histochemical GUS assays were performed in a staining solution containing 0.5 mg mL−1 5-bromo-4-chloro-3-indolyl glucuronide (X-Gluc) in 0.1 M Na2HPO4, pH 7.0, 10 mM Na2EDTA, 0.5 mM potassium ferricyanide/ferrocyanide, and 0.06 % Triton X-100 [58]. Samples were infiltrated under vacuum for 10 min and then incubated at 37 °C. After overnight incubation, the staining buffer was removed, and the samples were cleared in 70 % ethanol. All observations by light microscopy were made with the Olympus BX51 microscope system.
Chromatin immunoprecipitation-qPCR
Chromatin immunoprecipitation (ChIP)-qPCR experiments were performed following the protocol described previously [36], using 2-week-old light-grown wild-type and BZR1pro::BZR1-CFP (w2c) transgenic Arabidopsis seedlings. An affinity-purified anti-GFP polyclonal antibody was used to immunoprecipitate the BZR1 protein-DNA complex, and the precipitated DNA was analyzed by RT-qPCR using the SYBR@ Green reagent (Toyobo, Osaka, Japan). Results were presented as the ratio of the amount of DNA immunoprecipitated from BZR1-CFP samples to that of the control samples (wild-type). The UBC30 and PP2A genes were used as negative controls. The ChIP-qPCR experiments were performed three times, from which the means and standard deviations were calculated. The primer sequences for ChIP-qPCR are in Additional file 1: Table S1.
Protoplast transient expression assay
For transient expression of ERF49 by BZR1, the reporter plasmid ERF49pro::LUC and 35S::BZR1 effector or vector control were co-transformed into Arabidopsis protoplasts. Arabidopsis protoplasts were isolated as described previously [59]. The ERF49pro::LUC reporter gene construct contains the native sequence of the ERF49 promoter fused to luciferase (LUC). Luminescence activities of firefly and Renilla were measured using Dual-Luciferase Reporter Assay System reagent in a Modulus Luminometer/Fluorometer equipped with a luminescence kit (Promega). The relative reporter expression level was expressed as the LUC firefly/LUC Renilla ratio [60, 61].
Dual-luciferase reporter assay in tobacco
The pGreenII 0800-LUC fusion constructs carrying the promoters or the corresponding fragments of ERF49 and the pGreenII 62-SK::BZR1 construct were individually introduced into Agrobacterium tumefaciens strain GV3101. The Agrobacterium cells containing the 35S::BZR1 and the LUC fusion constructs were mixed at a ratio of 1:1 and infiltrated into Nicotiana benthamiana leaves [62]. After incubated for 3 days, the intensity of the firefly luciferase bioluminescence was measured using an imaging system (Xenogen IVIS 100, PerkinElmer). Luciferase activity was also detected using the Dual-Luciferase Reporter Assay System (Promega Corp., Fitchburg, WI, USA) according to the manufacturer’s protocol.
Yeast one-hybrid assay
BZR1 cDNA was inserted into the unique EcoRI and PspXI sites of the pJG45 vector. The ERF49 promoter and the corresponding fragments with intact or mutated BRRE cis-element variant were amplified from Arabidopsis and subcloned into the pLacZi vector [63] to drive lacZi reporter gene expression. The constructs were transformed into yeast strain EGY48. Positive transformants grown on the SD/-Trp/-Ura medium (Clontech, Mountain View, CA, USA) were transferred to the selection medium containing raffinose, galactose, and 5-Bromo-4-chloro-3-indolyl-b-D-galactopyranoside (Amresco, Solon, OH, USA) for blue color development. β-galactosidase activity was assayed by hydrolysis of ortho-nitrophenyl-β-D-galactopyranoside (ONPG), as well as measuring absorbance for the released ortho-nitrophenyl (ONP) compound on a spectrophotometer at 415 nm. Relevant PCR primer sequences are given in Additional file 1: Table S1.
Subcellular localization analysis
To determine the subcellular localization of gene products, the fusion construct (35S::ERF49-GFP) was transformed into tobacco leaf cells. Green fluorescence was observed under a confocal microscope (Leica TCS SP5, Wetzlar, Germany).
Expression analysis
The expression analysis of BZR1 and ERF49 was performed by the Arabidopsis eFP Browser (http://bar.utoronto.ca/efp/cgi-bin/efpWeb.cgi). The comparable developmental map was generated by typing appropriate parameters as described in original eFP Browser paper [64].
RNA-seq analysis
The ERF49 overexpression and Col plants were grown into 42 °C for 3 h, and about 100 mg leaves were used to extract total RNA. The 3 μg RNA was used to construct RNA-seq libraries and RNA-seq was repeated three times. Differentially expressed genes (DEGs) were identified with Fold Change ≥ 1.5 and adjusted p-values < 0.05. In addition, the published heat and BR treatment RNA-seq data were obtained [40, 41]. The GO analysis of genes downregulated in ERF49 overexpression lines and upregulated in BR and heat treatment plants were performed using agriGO v2.0 [65].
Availability of data and materials
All data generated or analyzed during this study are included in this published article, its supplementary information files and publicly available repositories. RNA-seq datasets are available from the NCBI sequence Read Archive BioProject (PRJNA891032).
Abbreviations
- ERF:
-
Ethylene responsive factor
- BZR1:
-
Brassinazole resistant 1
- BR:
-
Brassinosteroid
- HSF:
-
Heat shock factor
- HSP:
-
Heat-shock protein
- bzr1-1D:
-
Brassinazole-resistant 1-1D
- ROS:
-
Reactive oxygen species
- HSE:
-
Heat shock element
- PIF4:
-
Phytochrome interacting factor 4
- eBL:
-
Epi-brassinosteroid
- BRBT:
-
BR-regulated BZR1 target
- NLS:
-
Nuclear localization sequence
- BES1:
-
BRI1-EMS-Suppressor 1
- BIN2:
-
Brassinosteroid insensitive 2
- MYBL2:
-
Myeloblastosis family transcription factor-like 2
- DRE:
-
Dehydration-responsive element
- BRZ:
-
Brassinazole
- WS:
-
Wassilewskija
- ORF:
-
Open reading frame
- LUC:
-
Luciferase
- ChIP-chip:
-
Chromatin immunoprecipitation microarray
- OPNG:
-
Ortho-nitrophenyl-β-D-galactopyranoside
- ONP:
-
Ortho-nitrophenyl
- GO:
-
Gene Ontology
- EV:
-
Empty vector
References
Shekhawat K, Almeida-Trapp M, García-Ramírez GX, Hirt H. Beat the heat: plant- and microbe-mediated strategies for crop thermotolerance. Trends Plant Sci. 2022;27:802–13.
Li B, Gao K, Ren H, Tang W. Molecular mechanisms governing plant responses to high temperatures. J Integr Plant Biol. 2018;60:757–79.
Howarth CJ. Genetic improvements of tolerance to high temperature. In: Ashraf M, Harris PJC, editors. abiotic stresses: plant resistance through breeding and molecular approaches. New York: Howarth Press; 2005. p. 277–300.
Sakata T, Oshino T, Miura S, Tomabechi M, Tsunaga Y, Higashitani N, et al. Auxins reverse plant male sterility caused by high temperatures. Proc Natl Acad Sci U S A. 2010;107:8569–74.
Bi A, Wang T, Wang G, Zhang L, Wassie M, Amee M, et al. Stress memory gene FaHSP17.8-CII controls thermotolerance via remodeling PSII and ROS signaling in tall fescue. Plant Physiol. 2021;187:1163–76.
Li Z, Tang J, Srivastava R, Bassham DC, Howell SH. The transcription factor bzip60 links the unfolded protein response to the heat stress response in maize. Plant Cell. 2020;32:3559–75.
Rebeaud ME, Mallik S, Goloubinoff P, Tawfik DS. On the evolution of chaperones and cochaperones and the expansion of proteomes across the tree of life. Proc Natl Acad Sci U S A. 2021;118:e2020885118.
Sun X, Siri S, Hurst A, Qiu H. Heat Shock Protein 22 in physiological and pathological hearts: small molecule, large potentials. Cells. 2021;11:114–23.
Young JC, Hoogenraad NJ, Hartl FU. Molecular chaperones Hsp90 and Hsp70 deliver preproteins to the mitochondrial import receptor Tom70. Cell. 2003;112:41–50.
Reinle K, Mogk A, Bukau B. The Diverse functions of small heat shock proteins in the proteostasis network. J Mol Biol. 2022;434:167157. https://doi.org/10.1016/j.jmb.2021.167157.
Li SJ, Liu SC, Lin XH, Grierson D, Yin XR, Chen KS. Citrus heat shock transcription factor CitHsfA7-mediated citric acid degradation in response to heat stress. Plant Cell Environ. 2022;45:95–104.
Liu X, Meng P, Yang G, Zhang M, Peng S, Zhai MZ. Genome-wide identification and transcript profiles of walnut heat stress transcription factor involved in abiotic stress. BMC Genomics. 2020;21:474. https://doi.org/10.1186/s12864-020-06879-2.
Nolan TM, Vukašinović N, Liu D, Russinova E, Yin Y. Brassinosteroids: multidimensional regulators of plant growth, development, and stress responses. Plant Cell. 2020;32:295–318.
Wei Z, Li J. Brassinosteroids regulate root growth, development, and symbiosis. Mol Plant. 2016;9:86–100.
Krishna P. Brassinosteroid-mediated stress responses. J Plant Growth Regul. 2013;22:289–97.
Planas-Riverola A, Gupta A, Betegón-Putze I, Bosch N, Ibañes M, Caño-Delgado AI. Brassinosteroid signaling in plant development and adaptation to stress. Development. 2019;146:151894. https://doi.org/10.1242/dev.151894.
Kim TW, Guan SH, Sun Y, Deng ZP, Tang WQ, Shang JX, et al. Brassinosteroid signal transduction from cell-surface receptor kinases to nuclear transcription factors. Nat Cell Biol. 2019;11:1254–60.
Kim EJ, Russinova E. Brassinosteroid signalling. Curr Biol. 2020;30:294–8.
Wang ZY, Nakano T, Gendron J, He JX, Chen M, Vafeados D, et al. Nuclear-localized BZR1 mediates brassinosteroid-induced growth and feedback suppression of brassinosteroid biosynthesis. Dev Cell. 2002;2:505–13.
Luo XM, Lin WH, Zhu S, Zhu JY, Sun Y, Fan XY, et al. Integration of light- and brassinosteroid-signaling pathways by a GATA transcription factor in Arabidopsis. Dev Cell. 2010;19:872–83.
Yang Z, Yan B, Dong H, He G, Zhou Y, Sun J. BIC1 acts as a transcriptional coactivator to promote brassinosteroid signaling and plant growth. EMBO J. 2021;40:e104615.
Ibañez C, Delker C, Martinez C, Bürstenbinder K, Janitza P, Lippmann R, et al. Brassinosteroids dominate hormonal regulation of plant thermomorphogenesis via BZR1. Curr Biol. 2018;28:303–10.
Nakano T, Suzuki K, Fujimura T, Shinshi H. Genome-wide analysis of the ERF gene family in Arabidopsis and rice. Plant Physiol. 2006;140:411–32.
Licausi F, Ohme-Takagi M, Perata P. APETALA 2/Ethylene Responsive Factor (AP2/ERF) transcription factors: mediators of stress responses and developmental programs. New Phytol. 2013;199:639–49.
Cheng MC, Liao PM, Kuo WW, Lin TP. The Arabidopsis ETHYLENE RESPONSE FACTOR1 regulates abiotic stress-responsive gene expression by binding to different cis-acting elements in response to different stress signals. Plant Physiol. 2013;162:1566–82.
Yao Y, He RJ, Xie QL, Zhao XH, Deng XM, He JB, et al. ETHYLENE RESPONSE FACTOR 74 (ERF74) plays an essential role in controlling a respiratory burst oxidase homolog D (RbohD)-dependent mechanism in response to different stresses in Arabidopsis. New Phytol. 2017;213:1667–81.
Dhaubhadel S, Chaudhary S, Dobinson KF, Krishna P. Treatment with 24-epibrassinolide, a brassinosteroid, increases the basic thermotolerance of Brassica napus and tomato seedlings. Plant Mol Biol. 1999;40:333–42.
Sateesh K, Uday KD, Joan EK, Wilfred AK, Priti K. Brassinosteroid confers tolerance in Arabidopsis thaliana and Brassica napus to a range of abiotic stresses. Planta. 2007;225:353–64.
Mizoi J, Shinozaki K, Yamaguchi-Shinozaki K. AP2/ERF family transcription factors in plant abiotic stress responses. Biochim Biophys Acta. 2012;1819:86–96.
Meng X, Xu J, He Y, Yang KY, Mordorski B, Liu Y, et al. Phosphorylation of an ERF transcription factor by Arabidopsis MPK3/MPK6 regulates plant defense gene induction and fungal resistance. Plant Cell. 2013;25:1126–42.
Zhang G, Chen M, Li L, Xu Z, Chen X, Guo J, et al. Overexpression of the soybean GmERF3 gene, an AP2/ERF type transcription factor for increased tolerances to salt, drought, and diseases in transgenic tobacco. J Exp Bot. 2009;60:3781–96.
Sakuma Y, Liu Q, Dubouzet JG, Abe H, Shinozaki K, Yamaguchi-Shinozaki K. DNA-binding specificity of the ERF/AP2 domain of Arabidopsis DREBs, transcription factors involved in dehydration- and cold-inducible gene expression. Biochem Biophys Res Commun. 2002;290:998–1009.
Li XS, Zhang DY, Li HY, Wang YC, Zhang YM, Wood AJ. EsDREB2B, a novel truncated DREB2-type transcription factor in the desert legume Eremosparton songoricum, enhances tolerance to multiple abiotic stresses in yeast and transgenic tobacco. Plant Biol. 2014;14:1471–2229.
Hiratsu K, Matsui K, Koyama T, Ohme-Takagi M. Dominant repression of target genes by chimeric repressors that include the EAR motif, a repression domain, in Arabidopsis. Plant J. 2003;34:733–9.
Hiratsu K, Mitsuda N, Matsui K, Ohme-Takagi M. Identification of the minimal repression domain of SUPERMAN shows that the DLELRL hexapeptide is both necessary and sufficient for repression of transcription in Arabidopsis. Biochem Biophys Res Commun. 2004;321:172–8.
He JX, Gendron JM, Sun Y, Gampala SS, Gendron N, Sun CQ, et al. BZR1 is a transcriptional repressor with dual roles in brassinosteroid homeostasis and growth responses. Science. 2005;307:1634–8.
Yin Y, Vafeados D, Tao Y, Yoshida S, Asami T, Chory J. A new class of transcription factors mediates brassinosteroid-regulated gene expression in Arabidopsis. Cell. 2005;120:249–59.
Li JM, Nagpal P, Vitart V, McMorris TC, Chory J. A role for brassinosteroids in light-dependent development of Arabidopsis. Science. 1996;272:398–401.
Zhou XY, Li S, Xue HW. Brassinosteroids regulate the differential growth of arabidopsis hypocotyls through auxin signaling components IAA19 and ARF7. Mol Plant. 2013;6:887–904.
Darriere T, Jobet E, Zavala D, Escande ML, Durut N, de Bures A, et al. Upon heat stress processing of ribosomal RNA precursors into mature rRNAs is compromised after cleavage at primary P site in Arabidopsis thaliana. RNA Biol. 2022;19:719–34.
Clark NM, Nolan TM, Wang P, Song G, Montes C, Valentine CT, et al. Integrated omics networks reveal the temporal signaling events of brassinosteroid response in Arabidopsis. Nat Commun. 2021;12:5858.
Ren H, Wu X, Zhao W, Wang Y, Sun D, Gao K, et al. Heat shock-induced accumulation of the glycogen synthase kinase 3-like kinase BRASSINOSTEROID INSENSITIVE 2 promotes early flowering but reduces thermotolerance in Arabidopsis. Front Plant Sci. 2022;13:838062. https://doi.org/10.3389/fpls.2022.838062.
Albertos P, Dündar G, Schenk P, Carrera S, Cavelius P, Sieberer T, et al. Transcription factor BES1 interacts with HSFA1 to promote heat stress resistance of plants. EMBO J. 2022;41:e108664. https://doi.org/10.15252/embj.2021108664.
Sakuma Y, Maruyama K, Osakabe Y, Qin F, Seki M, Shinozaki K, et al. Functional analysis of an Arabidopsis transcription factor, DREB2A, involved in drought-responsive gene expression. Plant Cell. 2006;18:1292–309.
Peng X, Ma X, Fan W, Su M, Cheng L, Iftekhar A, et al. Improved drought and salt tolerance of Arabidopsis thaliana by transgenic expression of a novel DREB gene from Leymus chinensis. Plant Cell Rep. 2011;30:1493–502.
Schramm F, Larkindale J, Kiehlmann E, Ganguli A, Englich G, Vierling E, et al. A cascade of transcription factor DREB2A and heat stress transcription factor HsfA3 regulates the heat stress response of Arabidopsis. Plant J. 2008;53:264–74.
Yoshida T, Sakuma Y, Todaka D, Maruyama K, Qin F, Mizoi J, et al. Functional analysis of an Arabidopsis heat-shock transcription factor HsfA3 in the transcriptional cascade downstream of the DREB2A stress-regulatory system. Biochem Biophys Res Commun. 2008;368:515–21.
Lim CJ, Hwang JE, Chen H, Hong JK, Yang KA, Choi MS, et al. Over-expression of the Arabidopsis DRE/CRT-binding transcription factor DREB2C enhances thermotolerance. Biochem Biophys Res Commun. 2007;362:431–6.
Chen H, Hwang JE, Lim CJ, Kim DY, Lee SY, Lim CO. Arabidopsis DREB2C functions as a transcriptional activator of HsfA3 during the heat stress response. Biochem Biophys Res Commun. 2010;401:238–44.
Lee SJ, Kang JY, Park HJ, Kim MD, Bae MS, Choi HI, et al. DREB2C interacts with ABF2, a bZIP protein regulating abscisic acid-responsive gene expression, and its overexpression affects abscisic acid sensitivity. Plant Physiol. 2010;153:716–27.
Friedrich T, Oberkofler V, Trindade I, Altmann S, Brzezinka K, Lämke J, Gorka M, Kappel C, Sokolowska E, Skirycz A, et al. Heteromeric HSFA2/HSFA3 complexes drive transcriptional memory after heat stress in Arabidopsis. Nat Commun. 2021;12:3426. https://doi.org/10.1038/s41467-021-23786-6.
Liu J, Feng L, Gu X, Deng X, Qiu Q, Li Q, et al. An H3K27me3 demethylase-HSFA2 regulatory loop orchestrates transgenerational thermomemory in Arabidopsis. Cell Res. 2019;29:379–90.
Ye H, Li L, Guo H, Yin Y. MYBL2 is a substrate of GSK3-like kinase BIN2 and acts as a corepressor of BES1 in brassinosteroid signaling pathway in Arabidopsis. Proc Natl Acad Sci U S A. 2012;109:20142–7.
Solano R, Stepanova A, Chao Q, Ecker JR. Nuclear events in ethylene signaling: a transcriptional cascade mediated by ETHYLENE-INSENSITIVE3 and ETHYLENE-RESPONSE-FACTOR1. Genes Dev. 1998;12:3703–14.
Li J, Lease KA, Tax FE, Walker JC. BRS1, a serine carboxypeptidase, regulates BRI1 signaling in Arabidopsis thaliana. Proc Natl Acad Sci U S A. 2001;98:5916–21.
Hartley JL, Temple GF, Brasch MA. DNA cloning using in vitro site-specific recombination. Genome Res. 2000;10:1788–95.
Clough SJ, Bent AF. Floral dip: a simplified method for Agrobacterium-mediated transformation of Arabidopsis thaliana. Plant J. 1998;16:735–43.
Jefferson RA, Kavanagh TA, Bevan M. GUS fusions: β-glucuronidase as a sensitive and versatile gene fusion marker in higher plants. EMBO J. 1987;6:3901–7.
Yoo SD, Cho YH, Sheen J. Arabidopsis mesophyll protoplasts: A versatile cell system for transient gene expression analysis. Nat Protoc. 2007;2:1565–72.
Lin R, Ding L, Casola C, Ripoll DR, Feschotte C, Wang H. Transposase-derived transcription factors regulate light signaling in Arabidopsis. Science. 2007;318:1302–5.
Jiang Z, Xu G, Jing Y, Tang W, Lin R. Phytochrome B and REVEILLE1/2-mediated signalling controls seed dormancy and germination in Arabidopsis. Nat Commun. 2016;7:12377. https://doi.org/10.1038/ncomms12377.
Xie Y, Liu Y, Wang H, Ma X, Wang B, Wu G, et al. Phytochrome-interacting factors directly suppress MIR156 expression to enhance shade-avoidance syndrome in Arabidopsis. Nat Commun. 2017;8:348. https://doi.org/10.1038/s41467-017-00404-y.
Li G, Siddiqui H, Teng Y, Lin R, Wan XY, Li J, et al. Coordinated transcriptional regulation underlying the circadian clock in Arabidopsis. Nat Cell Biol. 2011;13:616–22.
Winter D, Vinegar B, Nahal H, Ammar R, Wilson GV, Provart NJ. An “electronic fluorescent pictograph” browser for exploring and analyzing large-scale biological data sets. PLoS One. 2007;2:e718. https://doi.org/10.1371/journal.pone.0000718.
Tian T, Liu Y, Yan H, You Q, Yi X, Du Z, et al. agriGO v2.0: a GO analysis toolkit for the agricultural community. Nucleic Acids Res. 2017;45:122–9.
Acknowledgements
We are grateful to Professor Zhi-yong Wang (Department of Plant Biology, Carnegie Institution of Washington) for helpful suggestions. We also thank Professor Lijia Qu (Peking University) for providing the p35S-SRDX vector, and RongCheng Lin (Institute of Botany, Chinese Academy of Sciences) for providing the pGreenII0800-LUC vector and pUC18-3HA vector. We also thank XinYu Zhang for advice and helpful on protoplast transient expression assay.
Funding
This work was supported by grants from the National Key Research and Development Program (2018YFD100070412, 2020YFA0907600), Hainan Yazhou Bay seed laboratory top-ranking project (B21HJ2005), and National Natural Science Foundation of China (32200444).
Author information
Authors and Affiliations
Contributions
XC and SZ designed the work; XC, YY, HL, HW, MF, and JZ performed research; XC, YL, ZL, and XL performed the analyses; HX, LZ, and GX contributed to the discussion and helped to structure the manuscript; XC and SZ wrote the original draft of the manuscript; HX, LZ, and GX managed the collaborative work and critically revised the manuscript. All authors read and approved the final manuscript.
Corresponding authors
Ethics declarations
Ethics approval and consent to participate
Not applicable.
Consent for publication
All authors read and approved the final manuscript.
Competing interests
The authors declare that they have no competing interests.
Additional information
Publisher’s Note
Springer Nature remains neutral with regard to jurisdictional claims in published maps and institutional affiliations.
Supplementary Information
Additional file 1: Figure S1.
Expression analysis of BZR1 and ERF49 in different tissues. Figure S2. Relative expression level of HSPs, HSFs and DREB2A in ERF49 transgenic lines. Figure S3. Bioinformatics analysis for binding of BZR1 to the ERF49 promoter. Figure S4. The analysis of hypocotyl elongation in the ERF49 transgenic plants with 2, 4-eBL treatment. Figure S5. Dominant-negative ERF49 increases thermotolerance in bzr1-1D. Figure S6. Thermotolerance analysis of ERF49 transgenic lines in the presence of 2, 4-eBL and BRZ. Table S1. Primers used in this study.
Additional file 2.
Detailed information of genes downregulated in ERF49 overexpression lines after heat treatment.
Additional file 3.
Detailed information of genes upregulated after heat treatment.
Additional file 4.
Detailed information of genes upregulated after BR treatment.
Additional file 5.
Detailed information of 30 overlap genes downregulated in ERF49 overexpression lines and upregulated after heat and BR treatment.
Additional file 6.
Combined raw data, with each figure on a spreadsheet.
Rights and permissions
Open Access This article is licensed under a Creative Commons Attribution 4.0 International License, which permits use, sharing, adaptation, distribution and reproduction in any medium or format, as long as you give appropriate credit to the original author(s) and the source, provide a link to the Creative Commons licence, and indicate if changes were made. The images or other third party material in this article are included in the article's Creative Commons licence, unless indicated otherwise in a credit line to the material. If material is not included in the article's Creative Commons licence and your intended use is not permitted by statutory regulation or exceeds the permitted use, you will need to obtain permission directly from the copyright holder. To view a copy of this licence, visit http://creativecommons.org/licenses/by/4.0/. The Creative Commons Public Domain Dedication waiver (http://creativecommons.org/publicdomain/zero/1.0/) applies to the data made available in this article, unless otherwise stated in a credit line to the data.
About this article
Cite this article
Chen, X., Xue, H., Zhu, L. et al. ERF49 mediates brassinosteroid regulation of heat stress tolerance in Arabidopsis thaliana. BMC Biol 20, 254 (2022). https://doi.org/10.1186/s12915-022-01455-4
Received:
Accepted:
Published:
DOI: https://doi.org/10.1186/s12915-022-01455-4