Abstract
During harvesting, storage, transportation, and processing, potato (Solanum tuberosum L.) tubers undergo greening after exposure to light, leading to the accumulation of toxic glycoside alkaloids, resulting in quality deterioration and economic losses. However, the underlying mechanisms are unclear. This study compared the transcriptome and proteome differences among four potato cultivars during the light-induced greening process, identifying 3,751 unique proteins (high confidence; ≥91.7%). The levels of enzymes involved in steroidal glycoalkaloid biosynthesis varied among the cultivars. In addition, coexpression network analysis of the transcriptomic data identified the transcription factor MYB113 (Soltu.DM.10G020780.1) as a potential positive regulator of steroidal glycoalkaloid biosynthesis. The dual-luciferase assay revealed that StMYB113 could bind to the promoters of steroidal glycoalkaloid biosynthesis-related genes and activate them. The transgenic lines overexpressing Solanum tuberosum L. Myb domain protein (StMYB113) exhibited greater mRNA abundance of these genes and elevated levels of steroidal glycoalkaloids. This study provided a theoretical basis for exploring the impact of light on the synthesis of solanine in potatoes.
Similar content being viewed by others
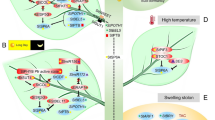
Introduction
Potatoes (Solanum tuberosum L.) are the fourth most important food crop, following maize, rice, and wheat, with a harvest area of 5.78 × 10^6 ha and a yield of 94.4 million tons in China (FAO 2021). As modified organs that develop from underground stolons, potato tubers are naturally incapable of performing photosynthesis due to the lack of exposure to direct sunlight. However, upon exposure to light, the amyloplasts located in the peripheral regions of the tubers transform into chloroplasts, leading to the accumulation of chlorophyll, the key pigment involved in photosynthesis; a process known as potato greening (Anstis et al. 1973; Zhu et al. 1984). However, the development of green tints on potato skin under field conditions prior to harvesting, attributable to chlorophyll formation upon sunlight exposure, is known as sunburn (Hardenburg 1964). Potatoes affected by sunburn are generally not retailed, as they are removed during grading. Typically, greening in potatoes refers to postharvest quality alterations (Dhalsamant et al. 2022) during storage, transportation, and processing (Tanios et al. 2018). Chlorophyll content in greening potatoes can reach 10 nm below the tuber periderm (Edwards et al. 1997). Chlorophyll is harmless, but the accumulation of bitter and toxic steroidal glycoalkaloids (SGAs) accompanies the greening process (Dhalsamant et al. 2022; Grunenfelder et al. 2006). Additionally, a green tuber is typically considered a defect since this hue is not the natural color of potatoes and is rejected by consumers (Mekapogu et al. 2016). Overall, greening is an unfavorable characteristic that reduces the marketability of potatoes, leads to increased waste at retail outlets, and ultimately impacts the pricing over time. According to the ‘United States Standards for Grades of Potatoes’ set by the US Department of Agriculture, an entire batch is subject to removal if it fails to meet the criteria for US grade #1, which includes instances where >5% of the potatoes in a batch exhibit greening (Dhalsamant et al. 2022). It is estimated that >10% of potatoes are wasted annually in Canada, partly due to greening (USDA 2018). The greening of potato tubers has been one of the crucial causes of potato quality deterioration and economic loss.
The biosynthesis pathway of glycoside alkaloids (Cárdenas et al. 2016; Itkin et al. 2013; Sawai et al. 2014), and the mechanism (Zhang et al. 2020) with influencing factors (Dhalsamant et al. 2022; Tanios et al. 2018) of potato tuber greening were studied. The accumulation of chlorophyll and glycoside alkaloids during greening is affected by various factors, such as the cultivar, temperature, and lighting during storage (Chang 2013; Edwards et al. 1997; Griffiths et al. 1994; Sabine. Tanios et al. 2020a, b, c). The maximum response wavelengths for chlorophyll synthesis were 475 and 625 nm, and those for SGAs were 430 and 650 nm (Petermann et al. 1985). Furthermore, greening is an enzymatic response that can be accelerated by temperature (Dhalsamant et al. 2022), and SGA content was enhanced with increasing temperatures from 5 to 23℃ (Rosenfeld et al. 1995). In addition, the synthesis of chlorophyll and glycoside alkaloids is believed to be independent (Edwards et al. 1998). Although chlorophyll and greening levels were significantly impacted by light sources, SGA accumulation was not markedly different during the greening of ‘Russet Burbank’ potato tubers (Olsen et al. 2017). Remarkable variations in SGA accumulation were identified between cultivars (Dale et al. 1993; Edwards et al. 1997). Therefore, it is essential to investigate the mechanisms of SGA biosynthesis during the tuber greening process. The genes related to stress response in potato tubers were conspicuously upregulated after exposure to light (Zhang et al. 2019). The accumulation rates of SGAs varied under different wavelengths of light (Okamoto et al. 2020). SGA biosynthesis can be induced by blue and red/far-red light exposure, suggesting the involvement of phytochrome. However, the effect of light on the accumulation of SGAs during greening has not been determined.
Transcription factors (TFs) regulate many physiological activities in plants, including photo-signaling, stress response, and synthesis of secondary metabolites (Chen et al. 2019; Li et al. 2021; Mahjoub et al. 2009; Xu et al. 2015). HY5 was involved in germination and photomorphogenesis in Arabidopsis (Zhang et al. 2011) and regulated the synthesis of glycoside alkaloids in tomato plants (Zhang et al. 2022). In potato plants, the expression of StMYBA1 was induced by light, which promoted the accumulation of anthocyanins in leaves (Zhao et al. 2023). This study used comparative transcriptome and proteome analyses to identify the differences among various potato materials during greening. The potential light-induced TF MYB113 was selected by dual-luciferase assay. The role of StMYB113 in the synthesis of solanine was verified by its overexpression and to further explore the regulatory mechanism of light on the synthesis of glycoside alkaloids in potatoes.
Materials and methods
Plant materials
The seeds of the cultivars ‘Innovator’, ‘11FF35-2’, ‘Stirling’, and ‘Huaen No. 2’ were sourced from the Key Laboratory of Potato Biology and Biotechnology, Ministry of Agriculture and Rural Affairs, Huazhong Agricultural University, Wuhan, China. The tubers were germinated in the dark at 28°C, planted in a 24 cm diameter bowl, and were grown under natural light conditions at 18–25°C in the greenhouse of the University. The planting was done on February 3, 2021, and harvesting on May 20 and June 9, 2021. Immediately after harvesting, the tubers were covered with a black shading cloth and stored at room temperature for two weeks before light treatment. Nicotiana benthamiana plants were subsequently grown in a growth chamber under 16 h light/8 h darkness conditions at 22℃. The transgenic strains were obtained from the College of Horticulture and Plant Protection, Henan University of Science and Technology, Luoyang, China (Zhang et al. 2023). The ‘Atlantic’ cultivar used for genomic DNA extraction was sourced from the Key Laboratory of Potato Biology and Biotechnology, Department of Agriculture and Rural Affairs, Huazhong Agricultural University.
Artificial induction of greening
Tubers of similar size and shape with no visible sprouting of the periderm, greening, or mechanical damage were selected for light treatment. They were weighed, those weighing 100±15 g were washed with clean water, and allowed to dry naturally in the dark. Then, half of the periderm area was wrapped with tin foil, and the sections covered were used as a control. The tubers were placed under a light intensity of 6000 lx at 25°C, uninterrupted, to induce greening. The tuber periderm was sampled at 0, 24, 48, 72, 96, and 120 h for follow-up tests. For sampling, potato tuber periderm and tissues 1.5–2.0 mm below the surface were removed, flash-frozen in liquid N2, ground into powder, and stored at -80°C for later use. Each tuber was a biological replicate, and random triplicate of samples were collected at each time point.
Measurement of chlorophyll and SAGs
The chlorophyll content was determined using a previous method (Wassie et al. 2019) with modifications. Briefly, 0.1 g of frozen powdered tissue sample was taken in a 2 mL centrifuge tube, 1.5 mL of 95% ethanol was added, the tube was sealed, and stored at room temperature under protection from light for 24 to 36 h to extract chlorophyll. Next, the OD665 and OD649 of the extract were measured. The following formula was used for calculating the total chlorophyll content [C] (mg/L):
Where W denotes the sample mass (g), and V represents the extract volume (mL).
The α-solanine and α-chaconine contents were determined employing a previous method (Itkin et al. 2011) with modifications. Briefly, 0.1 g of the sample powder was taken in a 1.5 mL centrifuge tube, 1.0 mL of extraction buffer (80% aqueous methanol containing 0.1% formic acid; v/v) was added, and vortexed for 30 s. The samples were extracted by ultrasonication for 60 min, vortexed for 30 s, and centrifuged at 12,000 rpm and 20°C for 10 min. Next, 25 μL of the supernatant was collected, added to a fresh centrifuge tube, diluted with 475 μL of ultrapure water containing 0.1% formic acid, and centrifuged at 14,000 rpm and 20°C for 15 min. Then, 150 μL of the supernatant was used for Liquid Chromatography-Mass Spectrometry (LC-MS) analysis. A Q Exactive Plus (Thermo Fisher Scientific, Waltham, USA) mass spectrometer and a 100 × 2.1 mm, 3 μm, Hypersil GOLD™ column (Thermo Fisher Scientific, USA) were used. The mobile phase A was an aqueous 0.1% formic acid solution, while the mobile phase B was an acetonitrile solution with 0.1% formic acid. The injection volume was 2 μL. The glycoside alkaloid content was calculated as the total α-solanine and α-chaconine contents. The samples were analyzed qualitatively based on the retention time and mass-to-charge ratio of the α-solanine and α-chaconine standards and analyzed quantitatively using the standard curve. The results are shown in the supplemental material (Fig. S1).
Extraction and preparation of periderm tissue proteins
Proteins were extracted and digested using the method of Isaacson et al. (2006) with modifications. All steps were conducted at 4°C or on ice. For extraction, 1 g of sample powder was resuspended in 10 × volume of TCA–acetone and precipitated overnight at -20°C. The precipitate was centrifuged at 12,000 rpm. The supernatant was discarded, and the pellet was washed thrice with precooled acetone until it turned white. The pellet was then dried in a fume hood overnight and resuspended in 500 μL lysis buffer (1% SDS, 100 mM Tris-HCl, 100 mM DTT; pH ± 8.0). The mixture was sonicated and incubated for 30 min. Before processing, the lysate was clarified by centrifugation at 12000 rpm for 10 min. The supernatant was transferred to a 1.5 mL microcentrifuge tube, after which the protein concentration was measured by Nano drop.
For trypsin digestion, 200 μg of protein from each tuber tissue sample was added in a 10 kD filter using the FASP method described previously (Wisniewski et al. 2009). After digestion, the samples were desalted with a MonoSpin C18 column (Shimadzu, Japan) and dried under a vacuum. The peptides were reconstituted in 0.1% formic acid aqueous solution.
Nonlabeled quantitative proteome and data analysis
Peptides were separated using nanoliter liquid chromatography with an Easy nLC1200™ System (Thermo Fisher Scientific, Waltham, USA). The loading volume of each peptide sample was 500 ng. The mobile phase A was a 0.1% formic acid aqueous solution, while the mobile phase B was an 80% acetonitrile solution containing 0.1% formic acid. The linear gradient was as follows: from 0 to 1 min, phase B increased from 3% to 8%; from 1 to 47 min, phase B increased from 8% to 22%; from 47 to 51 min, phase B increased from 22% to 26%; from 51 to 54 min, phase B increased from 26% to 35%; from 54 to 55 min, phase B increased from 35% to 100%, and the column was finally flushed at 100% for 5 min. The flow rate was 300 nL/min.
Protein profiling was performed using Q Exactive Plus high-resolution mass spectrometry (MS) (Thermo Fisher Scientific, Waltham, USA) with an electrospray ion source. The conditions were as follows: primary MS resolution, 70,000; ACG Target, 3e6; scanning range, 350–1,700; secondary MS resolution, 17,500; ACG Target, 1e5; and maximum IT, 50 ms. Twenty ions with the highest trigger intensities were selected for secondary MS (top 20), and the normalized collision energy (NCE) was 27.
The raw MS data files were exported to Proteome Discoverer v2.4 (Thermo Fisher Scientific, Waltham, USA) for analysis. They were searched using SEQUEST HT search engine built-in Thermo Scientific Proteome Discoverer (2.4) with a potato nucleocoding protein database containing 32,917 protein sequences and a chloroplast-coding protein database containing 86 protein sequences. The search range was set to phosphorylation of Thr, Ser, and Lys and N-terminal acetylation of the peptides. The precursor mass tolerance was 10 ppm, and the peptide mass tolerance was set to 0.05 Da. Only peptides with |q|<0.05 were accepted.
RNA extraction, cDNA synthesis and qRT-PCR
RNA from the sample powder was extracted using an RN33 Plant Total RNA Extraction Kit (Aidlab Biotechnologies Ltd., Beijing, China), following the instructions. The cDNA of the extracted RNA was obtained using G492 5 × All-In-One RT MasterMix Kit (Applied Biological Materials Inc., BC, Canada) by reverse transcription. The primers used for quantification were designed using the Primer-BLAST tool of NCBI (https://www.ncbi.nlm.nih.gov/), and the primer sequences are shown in Table S1. G891 2 × qPCR Master Mix (Applied Biological Materials) was used for qRT-PCR, performed as described in the kit instructions. The relative expression of the genes was calculated using EfIα as the internal reference gene by the 2-ΔΔCT method.
Bioinformatic analysis
RNAseq library preparation and sequencing were carried out by the Novogene Co. Ltd. (Beijing, China). Transcript quantification was performed using the Salmon software, and the transcripts were aligned with the updated DM1-3 v6.1 transcriptome (http://spuddb.uga.edu/dm_v6_1_download.shtml). Differentially expressed genes (DEGs) were identified using the DESeq2 tool. Specific benchmarks were established for identifying the significant DEGs, encompassing a false discovery rate (FDR) <0.05 and an absolute log2 (fold change) >1. Genes with increased expression were subjected to heatmap analysis, and the TFs were detected utilizing the ComplexHeatmap package in R. TBtools software was used to extract the promoter sequences of structural genes and analyze the cis-acting elements (Chen et al. 2020).
Dual-luciferase reporter assay
The sequences 2,000 bp upstream of the Coding sequences (CDSs) of the Cycloartenol synthase-like protein (CAS-like), CAS, Glycoalkaloid metabolism 6 (GAME6), GAME11, Hydroxy methylglutaryl CoA reductase (HMGR), steroidal alkaloid glycosyltransferase 3 (SGT3), sterol side chain reductase 2 (SSR2), and MYB113 genes were cloned from the ‘Atlantic’ gDNA and inserted into the reporter vector pGREEN0800II. The recombinant plasmids were used to transform the Agrobacterium tumefaciens strain GV3101 (pSoup) cells. Moreover, using ‘Huaen No. 2’ cDNA as a template, the CDSs (retaining the stop codon) of the candidate TFs were cloned and inserted into the linearized ph7lic-C-HA vector, which was subsequently employed to transform the GV3101 cells. The primers used for the promoter and CDS cloning are shown in Table S1. The injected tobacco leaves were sampled 60 h after injection, ground into powder in liquid nitrogen, and stored at -80°C.
Statistical analysis
The data were subjected to analysis of variance (ANOVA) using GraphPad Prism 8.4.2. Significant differences were detected using Dunnett’s multiple range test at the level of 5%.
Results
Phenotypic differences in tubers with different genetic backgrounds after light exposure
Alterations in the skin and metabolite contents of the tubers of the potato cultivars ‘Stirling’, ‘Huaen No. 2’, ‘Innovator’, and ‘11FF35-2’ were examined under alternating light and dark conditions over a 120 h span. Notably, the phenotypes differed among the cultivars. Specifically, ‘Stirling’ and ‘Huaen No. 2’ exhibited a greening of the epidermis, with the green tissue extending from the surface to the inner flesh as the period of light exposure extended. In contrast, ‘Innovator’ and ‘11FF35-2’ showed no notable greening (Fig. 1a). This distinct behavior underscores the former as varieties with heightened sensitivity to light-induced greening and termed greening-sensitive, while the latter emerges as the greening-resistant varieties. To elucidate the variations post-greening, the contents of chlorophyll and SGAs were assessed at 0, 24, 48, 72, and 120 h intervals (Fig. 1b). The chlorophyll content increased noticeably after 48 h of light exposure in all the samples. However, SGA accumulation varied, with that of ‘Stirling’ and ‘Huaen No.2’ increasing significantly and consistently post 72 h. However, SGA levels of ‘Innovator’ and ‘11FF35-2’ remained stable beyond this time point. These findings highlight the inherent phenotypic variability among tubers of different genotypes in response to light-induced greening.
Changes in phenotypes and metabolites content of four cultivars potato tubers under light conditions. (a) Changes in tubers of the four potato cultivars under light and dark conditions. Photographs of the phenotypes of tubers were collected daily to record changes continuously. (b) Light induction of chlorophyll and glycoalkaloid accumulation in the different cultivars. All the cultivars were exposed to light under 6,000 lx or darkness at 25°C for 0, 24, 48, 72, and 120 h. The data are the average of three replicates ± SD, and the various letters indicate the statistical significance of the differences between groups at P<0.05. DW, dryweight
Changes in the abundance of proteins related to SGAs and chlorophyll biosynthesis under light
Proteomic changes were analyzed during light-induced greening in the tubers of the four varieties to determine the variances in the greening process across the four genotypes. Samples were collected from the tubers of the four varieties subjected to light exposure at 0, 24, 48, 72, and 120 h. Total proteins were extracted from the green tuber tissues and subjected to laboratory-free quantitative proteomics. Using PD 2.4, the raw MS data were processed, and 3,751 unique proteins were identified by searching against the potato protein database, with 3,440 proteins having high confidence. Cluster analysis revealed remarkable alterations in protein abundance among the varieties (Fig. 2a and b).
Cluster diagram of the differentially accumulated proteins (DAPs) in the four potato cultivars. (a) Results of the principal component analysis (PCA) obtained using Proteome Discoverer 2.4; (b) Heatmap of the Label-free quantification analysis (LFQ) intensities of the DAPs in the treatment and control groups generated using Proteome Discoverer 2.4. (c) Statistical analysis of the DAPs in the four potato cultivars after light treatment. The up- and down-regulated proteins exhibited > 1.2-fold change and P <0.05
For a rigorous evaluation, differentially abundant proteins (DAPs) were assessed based on the following criteria: fold change (FC) >1.2 and P <0.05 at the time points studied (Fig. 2c). Differential protein expression patterns were observed throughout light exposure across the four potato varieties. Specifically, in ‘Stirling’, 639 DAPs comprising 519 up- and 120 down-regulated, while in ‘Huaen No. 2’, 616 DAPs, with 532 up- and 84 down-regulated, were identified. In contrast, ‘Innovator’ and ‘11FF35-2’ revealed fewer DAPs, with 458 and 529 DAPs, respectively; and varying numbers of up- and down-regulated proteins. The variations in protein expression patterns among the four potato varieties highlight the inherent genetic variability and underscore the complexities involved in the response of these plants to light-induced greening.
Kyoto Encyclopedia of Genes and Genomes (KEGG) enrichment analysis was conducted to delineate the potential metabolic pathways associated with these DAPs. As shown in Fig. 3, employing a P value <0.01, the DAPs from the four varieties were enriched in 23, 38, 27, and 23 metabolic pathways, respectively. The DAPs of all the cultivars were significantly enriched in ‘energy metabolism’, ‘photosynthesis’, ‘metabolism’, ‘photosynthesis proteins’, and ‘carbon fixation in photosynthetic organisms’. Notably, in the greening-sensitive varieties, a specific enrichment was observed in the steroid biosynthesis pathway (Fig. 3a, b), which is significant as steroids serve as vital precursors in the SGA metabolic pathway, implying that they may have a unique metabolic response contributing to their heightened sensitivity to light-induced greening. However, the metabolic pathways enriched by the DAPs after light exposure exhibited certain similarities among the potato varieties.
Tuber greening occurs when amyloplasts in the parenchyma cells beneath the epidermis turn into chloroplasts. This process involves the concurrent synthesis of chlorophyll and toxic SGAs. BLASTp alignment of the DAPs revealed 11 enzymes essential in the chlorophyll synthesis pathway and 10 critical enzymes in the glycoside alkaloid biosynthesis pathway (Fig. 4). Subsequently, quantitative real-time PCR (qRT-PCR) was performed to validate the proteomic results (Fig. S2). The heat maps illustrated that, during the greening process, the abundance of enzymes related to chlorophyll biosynthesis was consistent across the four plant varieties. However, there were notable disparities in the levels of enzymes associated with SGA biosynthesis in ‘Stirling’ and ‘Huaen No. 2’ after 48 h of light exposure, but not in ‘Innovator’ and ‘11FF35-2’. The expression levels of the genes encoding these enzymes after 0, 24, 48, and 72 h of light exposure evaluated by qRT-PCR (Fig. S2) indicated that the changes in the mRNA levels were consistent with the corresponding alterations in protein abundance (Fig. 4 and Fig. S2). These findings suggested that the variations in the enzyme abundance may be primarily attributed to differences in gene transcription, which in the genes for SGA synthesis could be the fundamental reason for the disparities in SGA accumulation across the four varieties post-light exposure.
TFs related to the synthesis of SGAs detected by RNA-seq
Due to a limitation in the throughput of the proteome, RNA-seq was conducted on the samples after 0 and 72 h of light exposure to further characterize the differences in gene expression and protein levels among these samples (Fig. S3). A scatter plot visualizing the distribution of mRNA and protein levels suggested a weak correlation, which was further confirmed by the remarkably low Pearson correlation coefficient of 0.236–0.342, suggesting that gene expression was not always positively associated with protein abundance in the periderm tissue of greening potato tubers. The DEGs were filtered to select those with similar trends of protein abundance and transcript expression. However, the number of such DEGs was meager and insufficient for further coexpression analysis. Weighted gene coexpression network analysis (WGCNA) was used to identify the highly correlated gene modules and hub genes related to SGA biosynthesis (Fig. S4). The samples were classified based on the duration of light exposure and genotype; nine gene coexpression modules were obtained (Fig. S4a). The dimgrey module contained 5,247 DEGs strongly positively correlated with light exposure duration and involved in SGA biosynthesis (Fig. S4b, c). The first 20 hub genes identified in this module were related mainly to photosystem formation or chlorophyll synthesis but not master regulators of SGAs (Fig. S4d).
Clustering analysis was subsequently employed to elucidate further the master regulators of SGAs. The heatmap constructed revealed that the genes associated with SGAs biosynthesis were exclusive to clusters 1 and 6 (Fig. 5a). After 72 h of light exposure, the expression of these genes was markedly elevated in all the varieties but was more pronounced in ‘Stirling’ and ‘Huaen No. 2’ than in ‘Innovator’ and ‘11FF35-2’. The genes related to chlorophyll biosynthesis were mainly in cluster 2. The transcriptomic analysis further confirmed the proteomic results, suggesting that chlorophyll and SGAs were synthesized independently in tubers during light exposure.
Co-expression analysis of structural genes and transcription factors in differential expression genes (DEGs). (a) Coexpression analyses of RNA-seq data. The red or green titles are the structural genes involved in synthesizing SGAs or chlorophyll. Those with pink, purple, or brown titles were consistently expressed in the same cluster. (b) Expression levels of structural and relevant TF-encoding genes in the four potato cultivars determined via RNA-seq/TPM
TFs are vital players in controlling the biosynthetic pathways of secondary metabolites, including SGAs, in potato plants (Cárdenas et al. 2016). Understanding the expression and activity of these TFs can provide insights into the molecular mechanisms that drive SGA accumulation, particularly under environmental conditions such as light exposure, which influence this process. Therefore, the relevant TF-encoding and structural genes in clusters 1 and 6 were coexpressed to determine in depth the mechanisms by which light orchestrates the synthesis of SGAs in potato tubers (Fig. 5b). These TFs belong to multiple families, such as the v-Myb myeloblastosis viral oncogene homolog (MYB) and Ethylene Response Factors (ERF), which are involved in photoresponse or alkaloid biosynthesis. However, candidate TFs need to be screened further.
Regulation of the structural genes by the candidate TFs
The promoter sequences of seven structural genes in the SGA biosynthesis pathway were cloned to ascertain whether the candidate TFs screened in the previous section could directly activate them. The promoter sequences possessed elements related to the external stimulus-response, such as the GATA motif (GATAGGG), the MYB binding site MRE (AACCTAA), and the MBS (CAACTG) (Fig. 6a). Based on the analysis of the cis-acting elements of the structural genes and the expression levels of candidate TFs (Fig. 5b), seven TFs from four different families: Soltu.DM.05G003170.1 | CO-like, Soltu.DM.04G002000.1 | CO-like, Soltu.DM.08G002010.1 | CO-like, Soltu.DM.12G001590.1 | GATA, Soltu.DM.10G023760.1 | WRKY, Soltu.DM.11G026620.2 | MYB, and Soltu.DM.10G020780.1 | MYB, were selected as potential candidates for regulating SGA synthesis and subjected to additional validation. The CDSs of the seven candidate TFs and the promoters of the seven structural genes related to SGA biosynthesis were subsequently cloned and inserted into the linear pH7li-C-HA or pGREENII 0800 vectors. The activation effects of the candidate TFs by binding to the promoters of the structural genes were analyzed using dual-luciferase reporter assays. The results indicated that, except for CO-like, all the other TFs bound to the promoters of the structural genes and activated them to varying degrees. Notably, StMYB113 (Soltu.DM.10G020780.1) bound to all the promoters except GAME6 (Soltu.DM.07G014190.1), which exhibited a particularly robust activation of the CAS-like (Soltu.DM.07G013460.1), CAS (Soltu.DM.04G026280.1), GAME11 (Soltu.DM.07G014170.1), and HMGR (Soltu.DM.02G022190.1) promoters. These results suggest that StMYB113 might be a critical TF regulating light-induced SGA accumulation (Fig. 6b).
Promoter analysis of structural genes and their transcriptional activities regulated by potential TFs. (a) Prediction of the presence of cis-acting elements in the promoters of genes involved in the biosynthesis of steroidal glycoalkaloids. (b) A transient dual-luciferase reporter assay confirmed the potential influence of the TFs on the expression of genes involved in the biosynthesis of steroidal glycoalkaloids. n ≥3, *P <0.05 determined by one-way analysis of variance (ANOVA), corrected for multiple comparisons using the Dunnett method. Pro-CAS-like, promoter sequence of cycloartenol synthase-like protein (Soltu.DM.07G013460.1); Pro-CAS, promoter sequence of cycloartenol synthase (Soltu.DM.04G026280.1); Pro-GAME6, promoter sequence of Cytochrome P450 (Soltu.DM.07G014190.1); Pro-HMGR, promoter sequence of hydroxy methylglutaryl CoA reductase (Soltu.DM.02G022190.1); Pro-SGT3, promoter sequence of steroidal alkaloid glycosyltransferase 3 (Soltu.DM.07G014160.1); Pro-SSR2, promoter sequence of sterol side chain reductase 2 (Soltu.DM.02G012480.1)
Overexpression of StMYB113 led to SGA accumulation in potato tubers
Considering the potentially crucial role of StMYB113 in light-induced SGAs accumulation, this study explored its functions by employing two previously obtained StMYB113-overexpressing lines (OE-14 and OE-15). Tubers of the transgenic and wild-type (WT) plants were harvested, stored in the dark, and sampled. qRT-PCR revealed that the expression levels of StMYB113 were remarkably greater in the tubers of the transgenic lines than in the WT plants. Similar were the expression patterns of StGAME11 (Soltu.DM.07G014170.1) and StGAME9 (Soltu.DM.01G031000.1) related to SGA biosynthesis (Fig. 7a). However, the expression of other SGA biosynthesis-related genes, such as CAS, GAME6, HMGR, SGT3, and SSR2, was not significantly higher in the transgenics (Fig. S5). Further analysis indicated that the SGA content in the flesh of tubers was considerably greater in those from the transgenic lines than the WT plants (Fig. 7b), although the periderm color was similar. However, the chlorophyll content was not conspicuously different between the transgenic lines and ‘Desiree’ plants (Fig. 7c and Fig. S5b). The variations in gene expression levels and metabolite contents between the transgenic and WT lines, coupled with the results of the transcriptional activation assays, suggest that StMYB113 may influence SGA biosynthesis in potatoes by modulating the expression of StGAME11 and other related genes.
Regulation of Solanum tuberosum L. Myb domain protein (StMYB113) gene on steroidal glycoalkaloids biosynthesis in potato tuber. (a) Gene expression analysis by qRT-PCR and (b) changes in the metabolite contents of potato tubers from two transgenic lines and Desiree plants. (c) The phenotype of the tubers from the transgenic lines or Desiree plants after harvest and storage in the dark. n = 3, *P <0.05, **P <0.01; one-way analysis of variance (ANOVA) was used, corrected for multiple comparisons using the Dunnett method
Discussion
Light-induced tuber greening characterized by the accumulation of chlorophyll and SGAs is a major cause of postharvest losses in potatoes. Studies over the past several decades have focused primarily on the intensity, duration, and spectral characteristics of light that induce tuber greening across different cultivars. Researchers have recently started elucidating the molecular mechanisms underlying the light-induced chlorophyll and SGAs accumulation in potatoes. For instance, an investigation of the effects of monochromatic light on the regulation of chlorophyll and glycoalkaloid accumulation in potato tubers reported that cryptochrome and phytochrome photoreceptors may play essential roles in this process (Okamoto et al. 2020). The variations in potato periderm and tuber maturity among different genotypes, along with their relationship with greening propensity, were analyzed, revealing that greening resistance was primarily associated with suberin content (Tanios et al. 2020a, b, c). Analysis of quantitative trait loci (QTLs) revealed that specific loci on chromosomes VII and X significantly contributed to the observed variations in potato tuber greening (Plich et al. 2020). Although these studies elucidated the molecular mechanisms underlying potato greening, the functional genes regulating the accumulation of SGAs during the greening process have not yet been identified.
Light-induced greening refers to the surface discoloration of potato tubers due to chlorophyll accumulation when potatoes are exposed to light during storage or processing (Dhalsamant et al. 2022; Sabine Tanios et al. 2020a, b, c). The greening process is often accompanied by the accumulation of toxic SGAs (Grunenfelder et al. 2006; Jadhav 1975; Zhang et al. 2020). The aggregation of chlorophyll and SGAs is not considered an interrelated phenomenon (Edwards et al. 1998), and the levels of SGAs in tubers after light exposure varied across potato varieties (Edwards et al. 1997; Griffiths et al. 1994). The results obtained in the present study are consistent with these conclusions (Fig. 1). Clustering analysis of 3440 high-confidence proteins revealed significant variations in abundance profiles across cultivars (Fig. 2). Notably, an unexpected clustering pattern emerged: the abundance groups of ‘Huaen No. 2’ at 0 clustered alongside ‘11FF35-2’ (Fig. 2b). This observation might be attributed to limitations inherent in the proteomic analysis method, specifically the throughput-related constraints of label-free quantification in the data-dependent acquisition (DDA) mode used in this study. Therefore, transcriptomic analysis was conducted, and the DEGs in each group were clustered individually (Fig. S6). The changes in protein abundance in the four varieties tested in this study demonstrated that the two pathways were independent of each other at the protein level (Fig. 4 and Fig. S2). These findings provide theoretical support for further efforts to analyze the mechanism of potato tuber greening after exposure to light.
Transcriptional regulation plays a vital role in the anabolism of secondary metabolites in plants. Previous studies on SGAs have focused mainly on their biosynthetic pathways (Cárdenas et al. 2019; Itkin et al. 2013; Itkin et al. 2011; Sonawane et al. 2022). In tomato, PIF3 from the bHLH TF family and HY5 from the bZIP TF family, which are involved in photomorphogenesis, regulated SGA biosynthesis (Wang et al. 2018; Zhang et al. 2022). The SGA contents changed, and proteomic data indicated that SGA accumulation during light-induced greening was a slow process that might be mediated by transcriptional regulation involved in the photo-response. The DEGs identified in this study by RNA-seq were clustered using WGCNA, and the TFs identified were annotated. In addition, trait association analysis of the differentially expressed TFs identified StPIF3 (Soltu.DM.01G041140.1) and StHY5 (Soltu.DM.08G011730.1) (Table S3 and Fig. S7), which are homologs of PIF3 and HY5 in potato, respectively, and were significantly correlated with SGA accumulation. HY5 was previously reported to be a positive regulator of the biosynthesis of SGAs. The transcript levels of GAMEs were reduced in HY5-silenced lines (Wang et al. 2018). However, in the present study, after exposure to light, the expression levels of Solanum tuberosum L. HY5 (StHY5) were down-regulated in ‘Innovator’ and ‘11FF35-2’, which presented a low accumulation of SGAs but remained stable in ‘Stirling’ and ‘Huaen No. 2’, which exhibited a high accumulation (Fig. 1 and Table S3), exhibiting a negative correlation with SGA accumulation (Table S3 and Fig. S7). This phenomenon might be attributed to the fact that greening is not a typical growth and development process in plants. However, further research is needed to explore the regulatory mechanism behind the differential expression of StHY5 among varieties.
TFs enhance or inhibit gene expression by binding to specific sequences upstream of the gene (Dubos et al. 2010). In potato and tomato, the ethylene-responsive factor AP2/ERF co-regulated SGAs’ biosynthesis along with MYC2???, and MYC2 played a regulatory role by binding to the G-box elements in the promoters of the downstream target genes (Cárdenas et al. 2016; Dombrecht et al. 2007). In this study, genomic DNA from the potato cultivar ‘Atlantic’ was used to amplify the promoter sequences of genes involved in the biosynthesis of SGAs. The cis-element analysis indicated the presence of other elements, such as MYB binding sites and G-box elements (Fig. 6a). A dual-luciferase reporter assay showed that StMYB113 remarkably activated the transcription of CAS-like, CAS, GAME11, HMGR, SGT3, and SSR2, which are involved in the biosynthesis of SGAs (Fig. 6b). In addition, the difference in metabolite contents in the tubers of the transgenic lines confirmed the involvement of StMYB113 in the biosynthesis of SGAs during tuber greening (Fig. 7b). The promoter of StMYB113 contains many cis-acting elements involved in light response, which indicated that StMYB113 regulated anthocyanin synthesis by responding to light in the potato cultivar ‘Desiree’ (Zhang et al. 2023). These results suggested that StMYB113 responds to light to regulate SGA biosynthesis during light-induced greening.
Conclusions
In summary, this study demonstrated that during potato tuber greening after light exposure, StMYB113 regulates the biosynthesis of SGAs by binding to the promoters of genes involved and activating their transcription. This research provides a new theory for studying the accumulation of SGAs during potato tuber greening. In addition, the biosynthesis of SGAs is a complex biochemical process regulated by several TFs. However, further efforts are required to investigate the mechanisms of transcriptional activation in this process.
Availability of data and materials
Raw data of proteomics and RNA-seq that support the findings of this study are not openly available due to reasons of sensitivity and are available from the corresponding author, Song (songbotao@mail.hzau.edu.cn), upon reasonable request. Data are located in controlled access data storage at College of Horticulture & Forestry Sciences of Huazhong Agricultural University.
References
Anstis PJP, Northcote DH. Development of chloroplasts from amyloplasts in potato tuber discs. New Phytol. 1973;72:449–63. https://doi.org/10.1111/j.1469-8137.1973.tb04394.x.
Cárdenas PD, Sonawane PD, Pollier J, Vanden Bossche R, Dewangan V, Weithorn E, et al. GAME9 regulates the biosynthesis of steroidal alkaloids and upstream isoprenoids in the plant mevalonate pathway. Nat Commun. 2016;7:10654. https://doi.org/10.1038/ncomms10654.
Cárdenas PD, Sonawane PD, Heinig U, Jozwiak A, Panda S, Abebie B, et al. Pathways to defense metabolites and evading fruit bitterness in genus Solanum evolved through 2-oxoglutarate-dependent dioxygenases. Nat Commun. 2019;10:5169. https://doi.org/10.1038/s41467-019-13211-4.
Chang HY. Light-induced glycoalkaloid and chlorophyll synthesis in potato tubers: cultivar differences and strategies for mitigation: University of California, Davis; 2013.
Chen L, Hu B, Qin Y, Hu G, Zhao J. Advance of the negative regulation of anthocyanin biosynthesis by MYB transcription factors. Plant Physiol Biochem. 2019;136:178–87. https://doi.org/10.1016/j.plaphy.2019.01.024.
Chen C, Chen H, Zhang Y, Thomas HR, Frank MH, He Y, et al. TBtools: an integrative toolkit developed for interactive analyses of big biological data. Mol Plant. 2020;13:1194–202. https://doi.org/10.1016/j.molp.2020.06.009.
Dale MFB, Griffiths DW, Bain H, Todd D. Glycoalkaloid increase in Solarium tuberosum on exposure to light. Ann Appl Biol. 1993;123:411–8. https://doi.org/10.1111/j.1744-7348.1993.tb04103.x.
Dhalsamant K, Singh CB, Lankapalli R. A review on greening and glycoalkaloids in potato tubers: potential solutions. J Agric Food Chem. 2022;70:13819–31. https://doi.org/10.1021/acs.jafc.2c01169.
Dombrecht B, Xue GP, Sprague SJ, Kirkegaard JA, Ross JJ, Reid JB, et al. MYC2 differentially modulates diverse jasmonate-dependent functions in Arabidopsis. Plant Cell. 2007;19:2225–45. https://doi.org/10.1105/tpc.106.048017.
Dubos C, Stracke R, Grotewold E, Weisshaar B, Martin C, Lepiniec L. MYB transcription factors in Arabidopsis. Trends Plant Sci. 2010;15:573–81. https://doi.org/10.1016/j.tplants.2010.06.005.
Edwards EJ, Cobb AH. Effect of temperature on glycoalkaloid and chlorophyll accumulation in potatoes (Solanum tuberosum L. Cv. King Edward) stored at low photon flux density, including preliminary modeling using an artificial neural network. J Agric Food Chem. 1997;45:1032–8. https://doi.org/10.1021/jf9607324.
Edwards EJ, Saint RE, Cobb AH. Is there a link between greening and light-enhanced glycoalkaloid accumulation in potato (Solanum tuberosum L.) tubers?. J Sci Food Agric. 1998;76:327–33. https://doi.org/10.1002/(SICI)1097-0010(199803)76:3<327::AID-JSFA934>3.0.CO;2-G.
Food and Agricultural Organization of the United Nations. https://www.fao.org/home/en (2021). Accessed 10 May 2023.
Griffiths DW, Dale MFB, Bain H. The effect of cultivar, maturity and storage on photo-induced changes in the total glycoalkaloid and chlorophyll contents of potatoes (Solanum tuberosum). Plant Sci. 1994;98:103–9. https://doi.org/10.1016/0168-9452(94)90153-8.
Grunenfelder LA, Knowles LO, Hiller LK, Knowles NR. Glycoalkaloid development during greening of fresh market potatoes (Solanum tuberosum L.). J Agric Food Chem. 2006;54:5847–54. https://doi.org/10.1021/jf0607359.
Hardenburg RE. Greening of potatoes during marketing—a review. Am Potato J. 1964;41:215–20. https://doi.org/10.1007/BF02855087.
Isaacson T, Damasceno CM, Saravanan RS, He Y, Catala C, Saladie M, et al. Sample extraction techniques for enhanced proteomic analysis of plant tissues. Nat Protoc. 2006;1:769–74. https://doi.org/10.1038/nprot.2006.102.
Itkin M, Rogachev I, Alkan N, Rosenberg T, Malitsky S, Masini L, et al. GLYCOALKALOID METABOLISM1 is required for steroidal alkaloid glycosylation and prevention of phytotoxicity in tomato. Plant Cell. 2011;23:4507–25. https://doi.org/10.1105/tpc.111.088732.
Itkin M, Heinig U, Tzfadia O, Bhide AJ, Shinde B, Cardenas PD, et al. Biosynthesis of antinutritional alkaloids in solanaceous crops is mediated by clustered genes. Science. 2013;341:175–9. https://doi.org/10.1126/science.1240230.
Jadhav SJ. Formation and control of chlorophyll and glycoalkaloids in tubers of Solanum tuberosum L. and evaluation of glycoalkaloid toxicity. Adv Food Res. 1975;21:307–54. https://doi.org/10.1016/s0065-2628(08)60093-2.
Li L, Li S, Ge H, Shi S, Li D, Liu Y, et al. A light-responsive transcription factor SmMYB35 enhances anthocyanin biosynthesis in eggplant (Solanum melongena L.). Planta. 2021;255:12. https://doi.org/10.1007/s00425-021-03698-x.
Mahjoub A, Hernould M, Joubès J, Decendit A, Mars M, Barrieu F, et al. Overexpression of a grapevine R2R3-MYB factor in tomato affects vegetative development, flower morphology and flavonoid and terpenoid metabolism. Plant Physiol Biochem. 2009;47:551–61. https://doi.org/10.1016/j.plaphy.2009.02.015.
Mekapogu M, Sohn HB, Kim SJ, Lee YY, Park HM, Jin YI, et al. Effect of light quality on the expression of glycoalkaloid biosynthetic genes contributing to steroidal glycoalkaloid accumulation in potato. Am J Potato Res. 2016;93:264–77. https://doi.org/10.1007/s12230-016-9502-z.
Okamoto H, Ducreux LJM, Allwood JW, Hedley PE, Wright A, Gururajan V, et al. Light regulation of chlorophyll and glycoalkaloid biosynthesis during tuber greening of potato S. tuberosum. Front Plant Sci. 2020;11:753. https://doi.org/10.3389/fpls.2020.00753.
Olsen NL, Brandt T, Price WJ. The Impact of retail light source on greening of russet gurbank potato tubers. Am J Potato Res. 2017;95:123–9. https://doi.org/10.1007/s12230-017-9616-y.
Petermann JB, Morris SC. The spectral responses of chlorophyll and glycoalkaloid synthesis in potato tubers (Solanum tuberosum L.). Plant Sci. 1985;39:105–10. https://doi.org/10.1016/0168-9452(85)90100-1.
Plich J, Zimnoch-Guzowska E, Tatarowska B, Sliwka J. Quantitative trait loci analysis of potato tuber greening. Mol Biol Rep. 2020;47:1713–22. https://doi.org/10.1007/s11033-020-05263-3.
Rosenfeld HJ, Sundell HA, Lea P, Ringstad M. Influence of packaging materials and temperature on the glycoalkaloid content of potato tubers. Food Res Int. 1995;28:481–4. https://doi.org/10.1016/0963-9969(96)81395-0.
Sawai S, Ohyama K, Yasumoto S, Seki H, Sakuma T, Yamamoto T, et al. Sterol side chain reductase 2 is a key enzyme in the biosynthesis of cholesterol, the common precursor of toxic steroidal glycoalkaloids in potato. Plant Cell. 2014;26:3763–74. https://doi.org/10.1105/tpc.114.130096.
Sonawane PD, Jozwiak A, Barbole R, Panda S, Abebie B, Kazachkova Y, et al. 2-oxoglutarate-dependent dioxygenases drive expansion of steroidal alkaloid structural diversity in the genus Solanum. New Phytol. 2022;234:1394–410. https://doi.org/10.1111/nph.18064.
Tanios S, Eyles A, Tegg R, Wilson C. Potato tuber greening: a review of predisposing factors, management and future challenges. Am J Potato Res. 2018;95:248–57. https://doi.org/10.1007/s12230-018-9648-y.
Tanios S, Tegg R, Eyles A, Thangavel T, Wilson C. Potato tuber greening risk is associated with tuber nitrogen content. Am J Potato Res. 2020;97:360–6. https://doi.org/10.1007/s12230-020-09786-0.
Tanios S, Thangavel T, Eyles A, Tegg RS, Nichols DS, Corkrey R, et al. Suberin deposition in potato periderm: a novel resistance mechanism against tuber greening. New Phytol. 2020;225:1273–84. https://doi.org/10.1111/nph.16334.
Tanios S, Eyles A, Corkrey R, Tegg RS, Thangavel T, Wilson CR. Quantifying risk factors associated with light-induced potato tuber greening in retail stores. PLoS One. 2020;15:e0235522-e. https://doi.org/10.1371/journal.pone.0235522.
U.S. Department of Agriculture: Potatoes and potato products annual 2018. https://www.fas.usda.gov/data/canada-potatoes-and-potato-products-annual-2018 (2018). Accessed 2 Oct 2018.
Wang CC, Meng LH, Gao Y, Grierson D, Fu DQ. Manipulation of light signal transduction factors as a means of modifying steroidal glycoalkaloids accumulation in tomato leaves. Front Plant Sci. 2018;9:437. https://doi.org/10.3389/fpls.2018.00437.
Wassie M, Zhang W, Zhang Q, Ji K, Chen L. Effect of heat stress on growth and physiological traits of alfalfa (Medicago sativa L.) and a comprehensive evaluation for heat tolerance. Agronomy. 2019;9:597. https://doi.org/10.3390/agronomy9100597.
Wisniewski JR, Zougman A, Nagaraj N, Mann M. Universal sample preparation method for proteome analysis. Nat Methods. 2009;6:359–62. https://doi.org/10.1038/nmeth.1322.
Xu W, Dubos C, Lepiniec L. Transcriptional control of flavonoid biosynthesis by MYB–bHLH–WDR complexes. Trends Plant Sci. 2015;20:176–85. https://doi.org/10.1016/j.tplants.2014.12.001.
Zhang H, He H, Wang X, Wang X, Yang X, Li L, et al. Genome-wide mapping of the HY5-mediated gene networks in Arabidopsis that involve both transcriptional and post-transcriptional regulation. Plant J. 2011;65:346–58. https://doi.org/10.1111/j.1365-313X.2010.04426.x.
Zhang W, Zuo C, Chen Z, Kang Y, Qin S. RNA sequencing reveals that both abiotic and biotic stress- responsive genes are induced during expression of steroidal glycoalkaloid in potato tuber subjected to light exposure. Genes (Basel). 2019;10:920. https://doi.org/10.3390/genes10110920.
Zhang C, Wu Y, Liu X, Zhang J, Li X, Lin L, et al. Pivotal roles of ELONGATED HYPOCOTYL5 in regulation of plant development and fruit metabolism in tomato. Plant Physiol. 2022;189:527–40. https://doi.org/10.1093/plphys/kiac133.
Zhang H, Zhao Z, Song B, Du P, Liu X. Light-induced ultrastructure changes of amyloplasts and effect of nitrogen fertilization on greening in potato tubers (Solanum tuberosum L.). Postharvest Biol Technol. 2020;168:111275.
Zhang Z, Zhou J, Zhao Y, Zhao X, Liu J, Liu J, et al. StMYB113 promotes anthocyanin biosynthesis in potato (Solanum tuberosum L.) Désirée tubers. Potato Res. 2023. https://doi.org/10.1007/s11540-023-09639-3.
Zhao X, Zhang H, Liu T, Zhao Y, Hu X, Liu S, et al. Transcriptome analysis provides StMYBA1 gene that regulates potato anthocyanin biosynthesis by activating structural genes. Front Plant Sci. 2023;14:1087121. https://doi.org/10.3389/fpls.2023.1087121.
Zhu YS, Merkle-Lehman DL, Kung SD. Light-induced transformation of amyloplasts into chloroplasts in potato tubers. Plant Physiol. 1984;75:142–5. https://doi.org/10.1104/pp.75.1.142.
Acknowledgements
Not applicable.
Funding
This research was supported by the National Key Research and Development Program of China (2022YFD1100201), the earmarked fund for CARS-09 and the HZAU-AGIS Cooperation Fund (SZYJY2023020).
Author information
Authors and Affiliations
Contributions
All authors contributed to the study conception and design. Plant materials were cultivated and harvested by Yunxia Cheng. Experiments, data collection and analysis were preformed by Shengxuan Liu, Enshuang Wang and Tiantian Liu. Transgenic lines were constructed by Xijuan Zhao and Huiling Zhang. The first draft of manuscript was written by Tengfei Liu, Botao Song and all authors commented on previous versions of the manuscript. All authors read and approved the final manuscript.
Corresponding authors
Ethics declarations
Ethics approval and consent to participate
Not applicable.
Consent for publication
Not applicable.
Competing interests
All authors declare that they have no competing interests.
Additional information
Publisher’s Note
Springer Nature remains neutral with regard to jurisdictional claims in published maps and institutional affiliations.
Supplementary Information
Additional file 1:
Fig. S1. Extracted ion chromatogram of (a) alpha-chaconine and (b) alpha-solanine. (c) The standard curves were made by standard samples from CHROMADEX company (#ASB-00019316-010 and #ASB-00003371-005). The fitting equations of alpha-chaconine and alpha-solanine are Y = -7.31183 × 10^6 + 352214 × X, R2 = 0.9957 and Y = -5.56196 × 10^6 + 242036 × X, R2 = 0.9955. Fig. S2. Validation of the expression of (a) chlorophyll and (b) steroidal glycoalkaloids metabolism pathway-related genes in different potato cultivars by qRT-PCR. Fig. S3. Comparison of the expression between transcriptomic (y-axis) and proteomic (x-axis) profiling. Log2 expression ratios were calculated from the expression of transcripts and proteins of (a) Innovator, (b) 11FF35-2, (c) Stirling, (d) Huaen No.2. Significant up-regulation genes (Log2FoldChange >1 and p.adj <0.05) were red dots, and down-regulation genes (Log2FoldChange <-1 and p.adj <0.05) were blue dots. Fig. S4. Weighted gene co-expression network analysis (WGCNA) of RNA-seq. (a) Co-expression modules were conducted by R programming language. The data at top is correlation coefficient, the data at below is p-value. (b) Expression of DEGs in dimgrey module. (c) The eigengene expression in dimgrey module. (d) Top 20 hub genes by WGCNA from module eigengene expression. Fig. S5. (a) Genes expression and (b) chlorophyll content in periderm tissue of potato tubers for 2 transgenic plants/Desiree under dark condition. Data represent means ± standard deviation (SD), n = 3, *P <0.05, **P <0.01, one-way ANOVA analyses, corrected for multiple comparisons using the Dunnett method. Fig. S6. Cluster diagram of DEGs in different potato cultivars by RNA-seq. Fig. S7. Trait association analysis on transcriptome data were conducted by R programming language.
Additional file 2:
Table S1. Primer list. Table S2. List of DEGs & DAPs. Table S3. The TPM of all annotation transcription factors and key enzymes.
Rights and permissions
Open Access This article is licensed under a Creative Commons Attribution 4.0 International License, which permits use, sharing, adaptation, distribution and reproduction in any medium or format, as long as you give appropriate credit to the original author(s) and the source, provide a link to the Creative Commons licence, and indicate if changes were made. The images or other third party material in this article are included in the article's Creative Commons licence, unless indicated otherwise in a credit line to the material. If material is not included in the article's Creative Commons licence and your intended use is not permitted by statutory regulation or exceeds the permitted use, you will need to obtain permission directly from the copyright holder. To view a copy of this licence, visit http://creativecommons.org/licenses/by/4.0/.
About this article
Cite this article
Liu, S., Cheng, Y., Zhao, X. et al. The transcription factor StMYB113 regulates light-induced greening by modulating steroidal glycoalkaloid biosynthesis in potatoes (Solanum tuberosum L.). HORTIC. ADV. 2, 7 (2024). https://doi.org/10.1007/s44281-023-00025-0
Received:
Revised:
Accepted:
Published:
DOI: https://doi.org/10.1007/s44281-023-00025-0