Abstract
Mechanically flexible transparent conductive electrodes (TCEs) with high optoelectronic performance are essential for flexible or wearable optoelectronic devices, which are currently receiving a considerable amount of attention. In this study, we investigate the structural, electrical, optical and mechanical properties of electrodeposited hierarchical silver network TCEs consisting of two layers of silver nanowires (AgNWs) and a silver micromesh. Hierarchical structures are known to improve the optoelectronic properties of network-type TCEs. To fabricate an electrodeposited hierarchical network, a AgNW solution is first spun onto a substrate to form randomly distributed AgNWs, and a silver micromesh is then formed on the AgNWs. Subsequently, silver is electrodeposited onto the hierarchical network. As a result of the electrodeposition, AgNW-AgNW and AgNW-silver micromesh contacts are effectively welded, and the dimensions of the AgNWs and the silver micromesh are optimized to maximize the figure of merit of the TCE. Furthermore, the electrodeposited hierarchical silver network shows excellent mechanical flexibility and much less degradation of its sheet resistance than that experienced by ITO upon repeated convex and concave bending. Its resulting optoelectronic and mechanically flexible performance is superior to that of commercialized ITO.
Graphical Abstract

Similar content being viewed by others
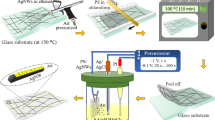
Avoid common mistakes on your manuscript.
1 Introduction
Transparent conducting electrodes (TCEs) that can be used for flexible or wearable optoelectronic devices must have low sheet resistance and high light transmittance, as well as mechanical flexibility [1,2,3,4,5,6,7,8,9,10,11,12,13]. Carbon nanotubes (CNTs), graphene, metal nanowires, and metal meshes are being studied as suitable materials for flexible TCEs [14,15,16,17,18,19]. The typical optoelectronic properties of mechanically flexible TCE materials, namely, the sheet resistance at 90% optical transmittance, are as follows. CNT: 84 Ω∕□ [20], graphene: 30 Ω∕□ [21], metal nanowires: 8–20 Ω∕□ [22], metal micromeshes: 5–10 Ω∕□ [23]. Among the abovementioned materials, metal micromeshes offer the best optoelectronic performance [24,25,26].
When network-type TCEs are employed in optoelectronic devices, charge carriers are present in the empty space of network-type TCEs such as CNTs, nanowires and micromesh [27,28,29]. Therefore, the relatively large empty space in the metal micromeshes may limit their application in optoelectronic devices. As a potential solution to this problem, nanoscale wires were added to a metal micromesh to form a hierarchical metal network [26]. A multiorder network has also been reported to be more suitable than a single-order network in ensuring lateral movement of charge carriers in the empty space of the network TCEs [30,31,32]
In this work, we improved the optoelectronic properties of a hierarchical silver network TCE by electrodeposition. An electrodeposited (ED) hierarchical silver network consisting of silver nanowires (AgNWs) and a silver micromesh was prepared. The silver micromesh provides main paths for electrical current flow, and the AgNWs provide local paths within the empty space of the micromesh. The electrodeposition of silver onto a hierarchical silver network enabled proper welding of AgNW-AgNW and AgNW-silver micromesh. The dimension of the silver micromesh was also adjusted to increase the figure of merit (FoM) of the hierarchical silver network TCE by electrodeposition. As a result of electrodeposition, the FoM of a hierarchical network TCE increased to 8.1 times before electrodeposition, which was 13.7 times that of a commercialized ITO thin film. Furthermore, the ED-hierarchical silver network showed much better mechanical flexibility than the ITO thin film upon repeated convex and concave bending. Therefore, the ED-hierarchical silver network is expected to be suitable for various optoelectronic device applications.
2 Experimental Section
2.1 Fabrication
The fabrication of an ED-hierarchical silver network TCE consists of three main steps: the first main step is to form randomly distributed AgNWs, the second main step is to form a silver micromesh on the AgNWs to form a hierarchical silver network, and the final main step is to electrodeposit silver onto the hierarchical silver network. Details of the processing steps are shown schematically in Fig. 1.
Schematic illustration of the processing step for an electrodeposited hierarchical silver network: a spin-coated AgNWs, b spin-coating of an acrylic resin layer, c a crack network template, d thermal evaporation of silver on the crack-network template, e deposition of the remaining silver micromesh onto the AgNWs to form a hierarchical silver network and f electrodeposition of silver onto the hierarchical silver network
AgNWs: To form randomly distributed AgNWs, AgNWs dispersed in ethanol (S32E, Nanopyxis) at a concentration of 0.05 wt. % were spun onto a PET substrate with a size of 2.5 cm × 2.5 cm by spin coating at 1000 rpm for 30 s followed by thermal annealing at approximately 120 °C for a few minutes to dry the solvent on a hot plate (Fig. 1a). The length and diameter of the AgNWs were approximately 25 \(\pm 5\) μm and 32 \(\pm 5\) nm, respectively.
Silver micromesh: To form a silver micromesh on the AgNWs, first, commercially available acrylic-based resin (Lubrizol Carboset CR735) was spin-coated on the AgNW-coated PET substrate at 3000 rpm for 30 s and dried at 120℃ (Fig. 1b). Volumetric shrinkage of the CR735 thin film during the drying process produced a randomly distributed and well-connected crack network (Fig. 1c). Silver with a thickness of 250 nm was then thermally evaporated onto the crack network (Fig. 1d). Subsequently, the sample was immersed in acetone and sonicated using an ultrasonicator (GT-X1, GT sonic) to remove the sacrificial crack network film. Therefore, the silver thin film deposited onto the crack network film was lifted off, and the silver deposited on the cracked region of the crack network film remained as a silver micromesh on the AgNWs to form a hierarchical silver network (Fig. 1e).
Electrodeposition of silver: Silver was electrodeposited onto a hierarchical silver network, which is a combination of AgNWs and a silver micromesh. In this process, a silver electrodeposition solution was prepared by dissolving 7 g of silver cyanide (Acros Organics, 180,230,500), 1.5 g of potassium carbonate (Alfa-Aesar, A16625), 15 g of potassium cyanide (Sigma‒Aldrich, 207,810), and 5 g of sodium thiosulfate pentahydrate (Alfa-Aesar, A17914) in 85 ml of DI water. A hierarchical silver network as a working electrode and a silver foil as a reference electrode were immersed in the silver electrodeposition solution under a constant current source of − 4.5 mA to obtain an ED-hierarchical silver network (Fig. 1f).
2.2 Characterization
The microstructures of the samples, including the AgNWs, a sacrificial crack-network template CR735 film, and hierarchical silver networks before and after electrodeposition of silver, were analyzed using field-emission scanning electron microscopy (FE-SEM, HITACHI SU8230). The optical transmittance of hierarchical network TCEs before and after electrodeposition of silver was analyzed by means of a UV‒vis spectrometer (UV-2600, Shimadzu) equipped with an ISR-2600 Plus integrating sphere using an employed substrate as a reference. The sheet resistance of the hierarchical network TCEs before and after the electrodeposition of silver was measured by a 4-point probe (FPP-40 K, Dasol Eng). The mechanical flexibility of an ED-hierarchical silver network TCE was tested by measuring its surface resistance after repeated convex and concave bending with a radius of curvature of 5 mm.
3 Results and Discussion
3.1 Microstructure
Figure 2a shows randomly distributed AgNWs on a PET substrate, corresponding to the schematic shown in Fig. 1a. The AgNWs do not have to form a network over a long distance because the AgNWs provide local paths within the empty space of a silver micromesh. The top and bottom widths of the U-shaped crack-network template (thickness = 3.15 μm) formed on the preformed AgNWs, corresponding to the schematic shown in Fig. 1c, were approximately 2.0 and 0.58 μm, respectively (Fig. 2b). The plane view of a sacrificial CR735 template formed on the AgNWs shows a well-connected crack network (Fig. 2c). A silver micromesh deposited on the cracked region of the sacrificial template shows a well-connected network formation over a long distance onto the preformed AgNWs (Fig. 2 (d)), corresponding to the schematic shown in Fig. 1e. The line width of the silver micromesh was approximately 0.55 μm, which is similar to the bottom width of the sacrificial crack-network template (Fig. 2d). Since the line width of the silver micromesh (~ 0.55 μm) was much larger than the diameter of AgNWs (~ 32 nm) (Fig. 2e), the silver micromesh was thought to provide primary paths for electrical current flow, and the AgNWs provide local paths within the empty space of the silver micromesh.
Figure 3 a shows an ED-hierarchical silver network after electrodeposition of silver for 30 s. The ED-hierarchical silver network shows clear AgNW-AgNW welding (Fig. 3b), which can ensure that the AgNWs provide concrete local paths within the empty space of the silver micromesh. The diameter of the AgNWs and the line width of the silver micromesh increased from ~ 32 to ~ 200 nm and from 0.55 to 0.72 μm upon electrodeposition for 30 s, respectively (Fig. 3b).
a-b Plane views of the hierarchical silver network electrodeposited for 30 s. The diameter of the AgNWs and the line width of the silver micromesh increased from ~ 32 to ~ 200 nm and from 0.55 to 0.72 μm after electrodeposition for 30 s, respectively. The ED-hierarchical silver network shows clear AgNW-AgNW welding, as indicted in the circle in Fig. 3b
3.2 Optoelectronic Properties
We investigated the optoelectronic properties of the AgNWs alone before discussing the variation in the sheet resistance and the optical transmittance of the hierarchical silver network TCE before and after the electrodeposition of silver. Figure 4a shows the sheet optical transmittance of the AgNWs, corresponding to Fig. 2a. The loss of optical transmittance of the AgNWs was only ~ 0.5%. The sheet resistance was infinite because the AgNWs did not form a continuous network. These AgNWs did not need to form a continuous network as long as they provided local current paths within the empty space of a silver micromesh in the hierarchical silver networks shown in Figs. 2d and 3b.
An increase in the diameter of the AgNWs and the line width in a silver micromesh upon electrodeposition improved the surface conductance of the hierarchical silver network. However, it also caused a loss of optical transmittance. Figure 4b and c shows the variation in the sheet resistance and the optical transmittance value at 550 nm of the hierarchical silver network TCEs as a function of the silver electrodeposition time. The hierarchical silver network had a sheet resistance of 8.3 Ω∕□ and an optical transmittance of 92%. The optical transmittance of the TCEs decreased linearly with the electrodeposition time at a rate as small as ~ 0.07%/s. On the other hand, the sheet resistance significantly decreased upon electrodeposition. Electrodeposition for as little as 10 s dramatically decreased the sheet resistance to 2.3 Ω∕□, with a loss of optical transmittance as low as 1%. After silver electrodeposition for 30 s, the sheet resistance of a hierarchical silver network TCE was only ~ 0.10 times the initial value, with a loss of optical transmittance of only 2.1%. These results demonstrate the effectiveness of the electrodeposition of silver onto a hierarchical network in improving the optoelectronic performance. The optical transmittance of the TCE remained almost constant over the measured wavelength region of the incident light (Fig. 4d) because the light passed through the empty space of the network, and light arriving on the silver surface of the AgNW was mostly reflected.
FoM generally determines the overall optoelectronic performance of a TCE and is expressed as the product of light transmittance and the reciprocal of sheet resistance, as shown below [32].
where T550 is the optical transmittance at a wavelength of 550 nm and RS is the sheet resistance.
Figure 5 shows the FoM values of ITO and the hierarchical network as a function of electrodeposition time. Even the nonelectrodeposited hierarchical network (FoM = 526 (Ω∕□)−1) showed a much higher FoM value than ITO (FoM = 311(Ω∕□)−1). As the electrodeposition time increased from 10, 20 and 30 s, the FoM increased to 1657, 2180, and 4263 (Ω∕□)−1, respectively. That is, we were able to adjust the optoelectronic performance of a hierarchical silver network by adjusting the electrodeposition time.
3.3 Mechanical Flexibility
One of the most important characteristics to be evaluated for application in flexible or wearable devices is mechanical flexibility. To evaluate the flexibility, a bending test was performed on ED-hierarchical silver networks formed on a PET substrate. Repetitive convex and concave bending with a radius of curvature of 5 mm was applied, and then the sheet resistance was measured. The sheet resistance of the commercialized ITO film increased to more than 2.5 times the initial value after 30 bending cycles. The ED-hierarchical silver network TCE with the best FoM of 4263(Ω∕□)−1 showed resistance changes of 7, 12, and 16% upon 50, 100, and 150 bending cycles, demonstrating that it had much better mechanical flexibility than ITO (Fig. 6).
4 Conclusion
We have demonstrated ED-hierarchical silver network TCEs consisting of AgNWs and a silver micromesh. We were able to significantly improve the optoelectronic performance of a hierarchical network TCE by the electrodeposition of silver. Electrodeposition for as short as 30 s dramatically decreased the sheet resistance of a TCE from 8.3 to 0.8 Ω∕□, with a relatively small loss in the optical transmittance as low as 2.1%. As a result, the FoM of the TCE increased to 8.10 times the initial value and was 13.7 times that of ITO. This significant improvement was achieved by adjusting the dimensions of each AgNW and the silver micromesh by electrodeposition. Furthermore, our ED-hierarchical network TCE showed much better mechanical flexibility than ITO. That is, the TCE showed a much smaller increase in the sheet resistance upon repeated convex and concave bending cycles than ITO. With these advantages, the ED-hierarchical silver network may be one of the best choices as a TCE for flexible or wearable optoelectronic devices.
References
Khan, A., Lee, S., Jang, T., Xiong, Z., Zhang, C., Tang, J., Guo, L.J., Wen-Di, L.: High-performance flexible transparent electrode with an embedded metal mesh fabricated by cost-effective solution process. Small 12(22), 3021–3030 (2016)
Jung, E.D., Nam, Y.S., Seo, H., Lee, B.R., Yu, J.C., Lee, S.Y., Kim, J.Y., Park, J.U., Song, M.H.: Highly efficient flexible optoelectronic devices using metal nanowire-conducting polymer composite transparent electrode. Electron. Mater. Lett. 11(5), 906–914 (2015)
Kumar, A., Zhou, C.: The race to replace tin-doped indium oxide: which material will win? ACS Nano 4(1), 11–14 (2010)
Kim, C.L., Jung, C.W., Oh, Y.J., Kim, D.E.: A highly flexible transparent conductive electrode based on nanomaterials. NPG Asia Mater. 9, e438 (2017)
Langley, D., Giusti, G., Mayousse, C., Celle, C., Bellet, D., Simonato, J.P.: Flexible transparent conductive materials based on silver nanowire networks: a review. Nanotechnology 24, 452001 (2013)
Shinde, M.A., Mallikarjuna, K., Noh, J., Kim, H.: Highly stable silver nanowires based bilayered flexible transparent conductive electrode. Thin Solid Films 660, 447–454 (2018)
Exarhos, G.J., Zhou, X.D.: Discovery-based design of transparent conducting oxide films. Thin Solid Films 515, 7025–7052 (2007)
Hecht, D.S., Hu, L., Irvin, G.: Emerging transparent electrodes based on thin films of carbon nanotubes, graphene, and metallic nanostructures. Adv. Mater. 23, 1482–1513 (2011)
Kim, J., Yun, A.J., Park, B., Kim, J.: Recent progress in carbon electrodes for efficient and cost-benign perovskite optoelectronics. Electron. Mater. Lett. 18, 232–255 (2022)
Gupta, R., Rao, K.D.M., Kulkarni, G.U.: Transparent and flexible capacitor fabricated using a metal wire network as a transparent conducting electrode. RSC Adv. 4, 31108–31112 (2014)
Yang, C., Merlo, J.M., Kong, J., Xian, Z., Han, B., Zhou, G., Gao, J., Burns, M.J., Kempa, K., Naughton, M.J.: All-solution-processed, scalable, self-cracking Ag network transparent conductor. Phys. Status Solidi A. 215, 1–6 (2018)
Sun, T., Metin Akinoglu, E., Guo, C., Paudel, T., Gao, J., Wang, Y., Giersig, M., Ren, Z., Kempa, K.: Enhanced broad-band extraordinary optical transmission through subwavelength perforated metallic films on strongly polarizable substrates. Appl. Phys. Lett. 102, 101114 (2013)
Park, J.S., Park, T.G., Park, J.S.: Characterization of Silver nanowire flexible transparent electrode with grid pattern formed via thermocompression. Electron. Mater. Lett. 17, 260–267 (2021)
Hsu, P.C., Wang, S., Wu, H., Narasimhan, V.K., Kong, D., Ryoung Lee, H., Cui, Y.: Performance enhancement of metal nanowire transparent conducting electrodes by mesoscale metal wires. Nat. Commun. 4, 2522 (2013)
Chee, S.S., Kim, H., Son, M., Ham, M.H.: Aspect ratio control of copper nanowire via solution process and its flexible transparent conductive electrode applications. Electron. Mater. Lett. 16, 404–410 (2020)
Hautcoeur, J., Castel, X., Colombel, F., Benzerga, R., Himdi, M., Legeay, G., Motta-Cruz, E.: Transparency and electrical properties of meshed metal films. Thin Solid Films 519, 3851–3858 (2011)
Song, T.B., Chen, Y., Chung, C.H., Yang, Y., Bob, B., Duan, H.S., Li, G., Tu, K.N., Huang, Y.: Nanoscale joule heating and electromigration enhanced ripening of silver nanowire contacts. ACS Nano. 8, 2804–2811 (2014)
Lee, S., Jang, J., Park, T., Park, Y.M., Park, J.S., Kim, Y.K., Lee, H.K., Jeon, E.C., Lee, D.K., Ahn, B., Chung, C.H.: Electrodeposited silver nanowire transparent conducting electrodes for thin-film solar cells. ACS Appl. Mater. Interfaces 12, 6169–6175 (2020)
Yang, M.K., Lee, J.K.: CNT/AgNW Multilayer electrodes on flexible organic solar cells. Electron. Mater. Lett. 16, 573–578 (2020)
Zhou, Y., Azumi, R.: Carbon nanotube based transparent conductive films: progress, challenges, and perspectives. Sci. Technol. Adv. Mater. 17, 493–516 (2016)
Bae, S., Kim, H., Lee, Y., Xu, X., Park, J.S., Zheng, Y., Balakrishnan, J., Lei, T., Ri Kim, H., Song, Y.. Il., Kim, Y.J., Kim, K.S., Özyilmaz, B., Ahn, J.H., Hong, B.H., Iijima, S.: Roll-to-roll production of 30-inch graphene films for transparent electrodes. Nat. Nanotechnol. 5, 574–578 (2010)
Hu, L., Kim, H.S., Lee, J.Y., Peumans, P., Cui, Y.: Scalable coating and properties of transparent, flexible, silver nanowire electrodes. ACS Nano 4, 2955–2963 (2010)
Kang, S., Arepalli, V.K., Yang, E., Lee, S., Wi, J.S., Yun, J.H., Song, S., Kim, K., Eo, Y.J., Cho, J.S., Gwak, J., Chung, C.H.: High performance and flexible electrodeposited silver mesh transparent conducting electrodes based on a self-cracking template. Electron. Mater. Lett. 18, 440–446 (2022)
Kulkarni, G.U., Kiruthika, S., Gupta, R., Rao, K.D.M.: Towards low cost materials and methods for transparent electrodes. Curr. Opin. Chem. Eng. 8, 60–68 (2015)
Lu, X., Zhang, Y., Zheng, Z.: Metal-based flexible transparent electrodes: challenges and recent advances. Adv. Electron. Mater. Lett. 7, 2001121 (2021)
Nguyen, V.H., Papanastasiou, D.T., Resende, J., Bardet, L., Sannicolo, T., Jiménez, C., Muñoz-Rojas, D., Nguyen, N.D., Bellet, D.: Advances in flexible metallic transparent electrodes. Small 18, 2106006 (2022)
Lee, S., Lee, J.S., Jang, J., Hong, K.H., Lee, D.K., Song, S., Kim, K., Eo, Y.J., Yun, J.H., Gwak, J., Chung, C.H.: Robust nanoscale contact of silver nanowire electrodes to semiconductors to achieve high performance chalcogenide thin film solar cells. Nano Energy 53, 675–682 (2018)
Lee, S., Jang, J., Cho, K.S., Oh, Y.J., Hong, K.H., Song, S., Kim, K., Eo, Y.J., Ho Yun, J., Gwak, J., Chung, C.H.: Determination of the lateral collection length of charge carriers for silver-nanowire-electrode-based Cu(In, Ga)Se2 thin-film solar cells. Sol. Energy 180, 519–523 (2019)
Chung, C.H.: Lateral photocurrent method for directly measuring the sheet resistance of a junction partner for a chalcogenide light absorber in a thin-film solar cell. Phys. Rev. Appl. 17, 034051 (2022)
Gao, J., Xian, Z., Zhou, G., Liu, J.M., Kempa, K.: Nature-inspired metallic networks for transparent electrodes. Adv. Funct. Mater. 28, 24 (2018)
Han, B., Peng, Q., Li, R., Rong, Q., Ding, Y., Akinoglu, E.M., Wu, X., Wang, X., Lu, X., Wang, Q., Zhou, G., Liu, J.M., Ren, Z., Giersig, M., Herczynski, A., Kempa, K., Gao, J.: Optimization of hierarchical structure and nanoscale-enabled plasmonic refraction for window electrodes in photovoltaics. Nat. Commun. 7, 12825 (2016)
Cho, K.S., Kang, S., Oh, Y.J., Park, J.S., Lee, S., Wi, J.S., Park, J.H., Song, S., Kim, K., Eo, Y.J., Yun, J.H., Gwak, J., Cho, J.S., Chung, C.H.: Hierarchical silver network transparent conducting electrodes for thin-film solar cells. ACS Appl. Electron. Mater. Lett. 4, 823–830 (2022)
Acknowledgements
This research was supported by the Basic Science Research Program through the National Research Foundation of Korea (NRF) that is funded by the Ministry of Science and ICT (Grant No. NRF-RS-2023-00239207, and NRF-2021R1A2C1005815)
Author information
Authors and Affiliations
Corresponding authors
Ethics declarations
Conflict of interest
We declare that this manuscript is original, has not been published before and is not currently being considered for publication elsewhere. The authors declare that they have no conflicts of interest to disclose that are relevant to the content of this article.
Ethical approval
All authors participated in (a) conception and design or analysis and interpretation of the data; (b) drafting the article or revising it critically for important intellectual content; and (c) approval of the final version.
Additional information
Publisher's Note
Springer Nature remains neutral with regard to jurisdictional claims in published maps and institutional affiliations.
Rights and permissions
Springer Nature or its licensor (e.g. a society or other partner) holds exclusive rights to this article under a publishing agreement with the author(s) or other rightsholder(s); author self-archiving of the accepted manuscript version of this article is solely governed by the terms of such publishing agreement and applicable law.
About this article
Cite this article
Yang, E., Kang, S., Jeong, S. et al. Electrodeposited Hierarchical Silver Network Transparent Conducting Electrodes with Excellent Optoelectronic Properties and Mechanical Flexibility. Electron. Mater. Lett. 20, 254–260 (2024). https://doi.org/10.1007/s13391-023-00453-0
Received:
Accepted:
Published:
Issue Date:
DOI: https://doi.org/10.1007/s13391-023-00453-0