Abstract
The nanomaterials silver nanoparticles accompanied magnetite impurity (Ag–Fe3O4) (AF) and cobalt nano ferrite/silver–magnetite (0.3 CoFe2O4/0.7Ag–Fe3O4) (CAF) nanocomposite were prepared by flash auto-combustion using urea as fuel. This is the first time preparing this new nanocomposite material. The as-prepared nanomaterials were characterized by X-ray diffraction pattern, atomic force microscopy, and Fourier transform infrared spectroscopy. This characterization affirmed that the nanoparticles were prepared in the nanoscale range with a cubic structure. The saturation magnetization of the CAF nanocomposite is 29-fold that of AF nanoparticles, and the coercivity of CAF is 19-fold that of AF. Moreover, a transformation from a soft hysteresis loop (AF) to a hard hysteresis loop for the CAF nanoscale makes CAF nanocomposite suitable for many technological applications, especially in a permanent magnet. Also, magnetic measurements showed that the operating frequency value of AF nanoparticles is very high in the radio wave region, and that of the CAF nanocomposite is an ultra-high frequency in the microwave S-band region. Unexpected behavior appeared for the antimicrobial activity of CAF nanocomposite. It was found that AF nanoparticles gave strong antimicrobial efficacy than CAF nanocomposite against the tested gram-positive and gram-negative bacteria. Thus, the AF and CAF nanomaterials are promising for many technological applications, especially biomedical ones for AF nanoparticles and a permanent magnet for CAF nanocomposite.
Similar content being viewed by others
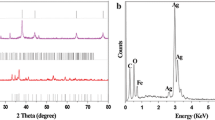
Avoid common mistakes on your manuscript.
1 Introduction
Nanotechnology is an important tool for tailoring materials with unique properties that can be applied in physics, chemistry, biology, and other sciences. Also, nanotechnology can address biomedical issues and solve problems in health and medicine [1, 2]. Physicist researchers are attracted to the synthesized new materials. They have studied the physical, magnetic, electrical, and optical properties of these unique materials with different structures and attractive features useful for applications in many fields [3,4,5,6,7]. Silver nanoparticles are an important material used to develop microbial resistance [8,9,10,11,12,13,14]. The addition of different compounds with different magnetic materials to silver nanoparticles has enhanced the material’s physical, magnetic, and antimicrobial properties. The study of magnetic nanomaterials is important because it helps us understand the behavior of the sample through various physical analyzes, and this will lead to the selection of appropriate applications.
Metallic nanoparticles of gold, copper, silver, zinc, titanium, and iron have various applications, especially in antimicrobial activities [15,16,17,18,19,20,21]. Furthermore, cobalt nano is a hard nanomaterial employed as a permanent magnet [22,23,24]. As a result, when it is added to silver magnetite nanoparticles as a nanocomposite, it improves their physical characteristics. They are prepared by various wet methods such as coprecipitation, sol–gel, flash, citrate, and oxalate [25,26,27]. The easiest method is the flash auto-combustion technique. This method has several advantages, such as short preparation time, high yield, and low cost. From previous studies, silver nanoparticles are known as a diamagnetic material. One can know that silver nanoparticles accompanied impurities had great attention [28]. Magnetite (Fe3O4) is one of the impurities added to silver nanoparticles and enhances the structural, magnetic, and antimicrobial properties [29].
Moreover, it occurs naturally on earth and crystallizes as a cubic system [29]. It is reported that silver coating on magnetite nanoparticles significantly reduces the magnetic characteristics of the magnetite nanopowder, which is consistent with our findings in the present study [30,31,32]. Thus, adding cobalt nano ferrite to silver magnetite nanoparticles in the present work increases the saturation magnetization by 29-fold. This is the first time preparing this new nanocomposite material to the authors' knowledge. Also, the novelty of the present work is studying the impact of adding a small concentration (0.3) of hard nanomaterial (cobalt) to a large concentration (0.7) of soft nanomaterial (silver–magnetite) to give a fascinating enhancement to the magnetic measurements. Moreover, unexpected behavior was studied when applying antimicrobial properties.
2 Experimental details
2.1 Sample preparation
First, the silver–magnetite nanoparticles (AF) were prepared using the flash method, as shown in Fig. 1. Nitrate salts, silver (8.49 g), and iron (20.2 g) were mixed with urea (10.01 g) after adding a small amount of distilled water to each of them. The mixture was heated at 500 °C until a fine powder was produced. The as-prepared sample was ground for one hour to be used in all analyses.
Second, the cobalt ferrite was prepared using the flash method. The starting materials were cobalt (14.55 g), and iron (40.4 g) nitrates with urea (20.03 g) mixed with a small amount of distilled water. Then the mixture was heated at 500 °C on a heater till a fine powder was prepared that was ground for an hour.
A stoichiometric ratio of 0.3 cobalt ferrite and 0.7 silver–magnetite nanoparticles was ground for 2 h to prepare the cobalt ferrite/silver–magnetite nanocomposite (CAF) ratio (30:70), as shown in Fig. 1.
2.2 Mechanism of synthesis of nanoparticles
Using chemical equations, the synthesis of silver magnetite and cobalt nano ferrite to produce the nanocomposite was as follows:
The nanoparticles were created with the assistance of the reducing agent urea at 500 °C. The studied samples were prepared using nitrate salts since they speed up the manufacturing process. The nanocomposite was then made by grinding a little cobalt nanoferrite (0.3) and silver magnetite (0.7).
2.3 Characterization
The thermal analyses were performed using a NETZSCH STA 409 C/CD apparatus. The X-ray diffraction pattern (XRD) was obtained with a DIANO Corporation apparatus using CuKα radiation with λ = 1.54 Å. The Fourier transform infrared (FTIR) spectroscopy analyses were obtained using a Jasco FTIR 300 E spectrometer. The morphology was examined by atomic force microscopy (AFM) using the Non-Contact Mode of a WET-SPM-9600. The magnetic properties were studied with a vibrating sample magnetometer (VSM; Lake Shore 7410).
2.4 Antimicrobial study
The antimicrobial activities of AF nanoparticles and CAF nanocomposites were studied in vitro using the disc diffusion method [33]. Bacillus subtilis (ATCC 6051), Streptococcus faecalis (ATCC 19433), and Streptococcus aureus (ATCC 12600) were gram-positive bacteria. Neisseria gonorrhea (ATCC 19424), Escherichia coli (ATCC 11775), and Pseudomonas aeruginosa (ATCC 10145) were gram-negative bacteria. Moreover, Aspergillus flavus (Link) and Candida albicans (ATCC 7102) fungi were studied against the investigated samples. All the tested bacteria and fungi against both samples were incubated at 30 °C for 24–48 h, and then the inhibition zone diameters were measured.
3 Results and discussions
3.1 Thermal analyses
Figure 2a presents the differential scanning calorimetry (DSC) curve of AF nanoparticles and shows that two peaks appeared. The first is an exothermic peak at 250 °C due to the dehydration of the sample. However, the second peak is an endothermic one that appeared at 380 °C due to the complete formation of the sample. Figure 2b shows the thermogravimetric analysis (TG), differential thermogravimetry (DTG), and differential thermal analysis (DTA) curves for AF nanoparticles. The TG curve indicated a weight loss at around 99 °C–224 °C due to the combustion of nitrate salts with urea [34]. Above 416 °C, the sample exhibited the formation of the nano sample as demonstrated in the XRD analysis. Accompanying the decrease of the TG curve, an endothermic peak appeared at 100 °C–200 °C in the DTG curve due to the combustion of nitrate salts and the fuel (urea). Above the second endothermic peak, the sample becomes stable and exhibits the formation of the nano sample at higher than 400 °C. Thus, the investigated sample was prepared at 500 °C.
3.2 XRD study
Figure 3 shows the XRD analyses of AF and CAF nanocomposite. ICDD cards indexed the diffraction peaks of AF nanoparticles for silver nanoparticles (Ag; 04-004-6436) and magnetite (Fe3O4; 01-084-9338). CAF nanocomposite was investigated with ICDD cards for Ag, Fe3O4, and cobalt ferrite (CoFe2O4; 04-005-7078). The XRD analysis revealed that the investigated sample exhibited a cubic structure, as shown in Fig. 3. The crystallite size values of the investigated samples are shown in Table 1. The crystallite size was calculated using the basic Scherrer equation [35,36,37,38,39,40,41]:
where K = 0.9 is the shape factor, λ = 1.54 Å is the wavelength of CuKα radiation, and β is the full width at half maximum. The crystallite sizes of the investigated samples were in the nanoscale range.
3.3 FTIR study
The FTIR spectra for the investigated samples are displayed in Fig. 4, and the peak values are reported in Table 2. The spectrum consisted of two main absorption peaks, peaks 1 and 2. These peaks were attributed to the tetrahedral A-site (peak 2) and the octahedral B-site (peak 1). Peak 3 was assigned to the liberation of water. Moreover, peak 4 was assigned to the stretching C–N vibrational band that appeared due to the combustion of metal nitrates during the preparation of the investigated samples. Also, peaks 5 and 6 were assigned to the vibrational C=O group, while peak 7 was assigned to the stretching vibrational band of the C–O–C group. Finally, peaks 8 and 9 were due to the stretching vibrations of the OH group. All the FTIR analyses were in good agreement with previous studies [42,43,44,45].
3.4 AFM study
Figure 5a–d shows the morphology of CAF nanocomposite and AF nanoparticles obtained by AFM. The agglomeration shown in the micrograph is due to the absence of adding surfactant during the preparation method [36]. This agglomeration was due to the magnetostatic interaction of the nanoparticles. Table 1 lists the values for particle size of the investigated samples estimated from the histogram deduced from the AFM micrograph, as shown in Fig. 6a, c. One can observe that by adding cobalt nano ferrite to silver–magnetite nanoparticles (CAF), the particle size increased more than that of silver–magnetite nanoparticles (AF).
Moreover, the particle size value estimated from AFM is larger than the crystallite size estimated from the XRD analysis. This was attributed to the particle size resulting from the agglomeration of small crystallites [46]. Figure 6b, d shows the surface roughness, the values reported in Table 1 and showed that the roughness increased by adding cobalt to silver–magnetite nanoparticles (CAF) than that of AF nanoparticles. This may be due to the multiphase that appeared in the CAF nanocomposite. Finally, the AFM morphology and XRD analysis results indicate that the particle sizes of the investigated samples were in the nano-size range.
3.5 Magnetic study
Figure 7 shows the magnetic hysteresis loop measurements obtained by vibrating sample magnetometer (VSM) for AF and CAF nanocomposite at room temperature. The magnetic parameters were calculated and reported in Table 3. One can observe that an attractive enhancement happened by adding a small concentration of cobalt ferrite (CoFe2O4) to silver–magnetite (Ag–Fe3O4) nanoparticles. The saturation magnetization, Ms, of CAF nanocomposite increased 29-fold over that of AF nanoparticles that have been used in many technological applications. There is a correlation between the high Ms and the high antimicrobial properties, as discussed in Sect. 3.7. Also, the coercivity, Hc, of CAF nanocomposite increased 19.8-fold over AF due to the large increase in magnetocrystalline anisotropic constant k (568.9-fold) over that of AF nanoparticles. The magnetocrystalline anisotropic constant (k) is calculated from the following relation [47,48,49,50]:
Moreover, this large increase of coercivity for CAF nanocomposite that transforms the hysteresis loop system from soft to hard ferrite is an attractive result with many potential applications, such as in permanent magnets and magnetic targeting and separators. Table 3 lists the value of the squareness R, calculated from the following equation [51,52,53,54]:
where Mr is the remnant magnetization. The type of interaction between nanoparticles was determined from the squareness value. The present study identified the interaction between nanoparticles for both samples as magnetostatic because the squareness value was less than 0.5 (R < 0.5).
Figure 8 shows the magnetic susceptibility (χ = dM/DH) against the applied field for the AF nanoparticles and CAF nanocomposite. The χ of CAF is larger by 68.4-fold than that of AF nanoparticles due to the large increase in the Ms of CAF nanocomposites. Moreover, the width of the CAF nanocomposite is wider than that of AF nanoparticles due to the very large coercivity of the CAF nanocomposite. Also, a multiphase regime appeared in Fig. 8 for CAF nanocomposite due to the inefficient exchange coupling between hard and soft magnetic phases of cobalt ferrite and silver–magnetite.
3.6 High-frequency application
Figure 9 shows the operating frequency response of the investigated samples calculated from the magnetic measurements. One can observe that the CAF nanocomposite had a very large operating frequency compared with AF nanoparticles. Many factors affect operating frequency ranges, such as the magnetization of the nanomaterials and the geometry of the devices. It is important to check the performance of a device by knowing the value of the operating frequency. The operating frequency (ω) was calculated using the following relationship [55, 56]:
where M is the magnetization of the investigated samples, and γ is the gyromagnetic ratio (γ = 2.8 MHz/G). As a result, the very high value of the operating frequency of the AF nanoparticles is calculated as 0.11 GHz (113.9 MHz), which is in the radio wave region. However, the ultra-high value of the operating frequency of CAF nanocomposite is 3.3 GHz which is in the microwave S-band region [55, 56].
3.7 Antimicrobial study
Figures 10, 11, and 12 show the results of in vitro antimicrobial studies for AF nanoparticles and CAF nanocomposites, and Table 4 lists the inhibition zone diameters of the investigated samples against gram-positive bacteria (Bacillus subtilis, Streptococcus faecalis, Streptococcus aureus) and gram-negative bacteria (Neisseria gonorrhea, Escherichia coli, and Pseudomonas aeruginosa). Moreover, Aspergillus fulvus (Link) and Candida albicans fungi were studied but exhibited no activity against the investigated samples. Table 4 reports the values of the inhibition zone parameters of AF nanoparticles, CAF nanocomposite, ampicillin (antibacterial agent), and amphotericin B (Antifungal agent) against the tested bacteria and fungi. One can know that there is a correlation between the increase in magnetization and the increase in antimicrobial properties [57]. However, unexpected behavior appeared in antimicrobial properties for CAF nanocomposite (higher magnetization) that showed lower antimicrobial properties than that of AF nanoparticles (lower magnetization). This may be attributed to the larger coercivity of the CAF nanocomposite than that of AF nanoparticles. The AF nanoparticles showed stronger activity against the tested bacteria than the CAF nanocomposite. This was attributed to a larger concentration of silver nanoparticles in AF nanoparticles than in the CAF nanocomposite, and, as is known, silver nanoparticles are the most toxic nanomaterial against the tested bacteria [58]. Moreover, both samples showed strong activity against the tested bacteria due to damage to the DNA in the cell membranes of the tested bacteria [59,60,61]. Finally, the investigated samples showed strong antimicrobial activities and are recommended as antibacterial nanomaterials for biomedical applications.
4 Conclusion
In conclusion, the impact of adding 0.3 cobalt nano ferrite (CoFe2O4) to 0.7 silver–magnetite (Ag–Fe3O4) nanoparticles was studied and compared to Silver–magnetite (Ag–Fe3O4) nanoparticles. They were prepared successfully by the flash auto-combustion method. Structural analyses showed the formation of the cubic structure of the nanocomposite from XRD analysis. Also, the particle morphology was nanosized. From the results of the magnetic measurements, the CAF nanocomposite showed larger Ms, Hc, Mr, k, and χ than the AF nanoparticles. Also, magnetic measurements showed that the value of the operating frequency of AF nanoparticles is very high frequency in the radio wave region, and that of CAF nanocomposite is an ultra-high frequency in the microwave S-band region. Thus, these results make CAF nanocomposites a promising nanomaterial in many applications. Although only a small concentration of cobalt ferrite (0.3) was added to silver–magnetite nanoparticles, an attractive result happened, especially in magnetic measurements that indicate a variety of technological applications. The suggested applications for CAF nanocomposite are as a permanent magnet due to the large coercivity and in magnetic targeting and separators due to the large saturation magnetization. Both materials have antimicrobial applications. The antimicrobial activities were studied against bacteria and showed a stronger activity of AF nanoparticles than that of CAF nanocomposite. However, no activity appeared against the tested fungi for both materials. Thus, the investigated samples could be used as alternative antibacterial agents against various drugs.
Data availability
The data that support the findings of this study are available on request from the corresponding author.
References
T.K. Rao, C.H.J. Rao, I.V.K. Viswanath, Y.L.N. Murthy, Anti-microbial activity of nanosilver ferrite composite. IJIRSET (2015). https://doi.org/10.15680/IJIRSET.2015.0409092
M.A. Sayed, H.K. Abdelsalam, A.A.H. El-Bassuony, Antimicrobial activity of novel spinel nanoferrites against pathogenic fungi and bacteria, World. J. Microbiol. Biotechnol. 36, 25 (2020). https://doi.org/10.1007/s11274-020-2803-x
A.A.H. El-Bassuony, Enhancement of structural and electrical properties of novelty nanoferrite materials. J. Mater. Sci. 28, 14489–14498 (2017). https://doi.org/10.1007/s10854-017-7312-9
A.A.H. El-Bassuony, H.K. Abdelsalam, W.M. Gamal, Influence of elastic and optical properties on AgFeO2 and AgCrO2 delafossite to be applied in high frequency applications. JOM (2022). https://doi.org/10.1007/s11837-022-05170-x
H.K. Abdelsalam, Enhancing the structural and spectroscopic properties of Cr3+ ion-doped Ni/Cd/Zn nanoferrite to be applied to industrial applications. J. Supercond. Novel Magn. 31, 4063–4077 (2018). https://doi.org/10.1007/s10948-018-4689-5
F. Xue, Z. Liu, Yi. Su, K. Varahramyan, Inkjet-printed silver source/drain electrodes for low-cost polymer thin-film transistors. Micro- electron. Eng. 83, 298 (2006)
A.A.H. El-Bassuony, A comparative study of physical properties of Er and Yb nanophase ferrite for industrial application. J. Supercond. Novel Magn. 31, 2829–2840 (2018). https://doi.org/10.1007/s10948-017-4543-1
R.J.B. Pinto, P.A.A.P. Marques, C.P. Neto, T. Trindade, S. Daina, P. Sadocco, Antibacterial activity of nanocomposites of silver and bacterial or vegetable cellulosic fibers. Acta Biomater. 5, 2279–2289 (2009)
S. Gamerith, A. Klug, H. Scheiber, U. Scherf, E. Moderegger, E.J.W. List, ‘Direct Inkjet printing of Ag-Cu nanoparticles and Ag precursor based electronics for OFET application.’ Adv. Funct. Mater. 17, 3111 (2007)
A.A.H. El-Bassuony, Effect of Al addition on structural, magnetic, and antimicrobial properties of Ag nanoparticles for biomedical applications. JOM 72, 1154–1162 (2020). https://doi.org/10.1007/s11837-019-03784-2
Y. Lu, K. Chou, A simple and effective route for the synthesis of nano-silver colloidal dispersions. J. Chin. Inst. Chem. Eng. 39, 673–678 (2008). https://doi.org/10.1016/j.jcice.2008.06.005
E.S. Anooj, S.J. Sreelekshmi, S.T. Gopukumar, P.K. Praseetha, Evaluation of the zinc ferrite nanoparticles for bio-applications. Int. J. Pharm. Sci. Rev. Res. 46, 22–26 (2017)
A.A.H. El-Bassuony, H.K. Abdelsalam, Synthesis, characterization and antimicrobial activity of AgFeO2 delafossite. J. Mater. Sci. 29, 11699–11711 (2018). https://doi.org/10.1007/s10854-018-9268-9
K. Jyoti, M. Baunthiyal, A. Singh, Characterization of silver nanoparticles synthesized using Urtica dioica Linn. leaves and their synergistic effects with antibiotics. J. Radiat. Res. Appl. Sci. 9(3), 217–227 (2016). https://doi.org/10.1016/j.jrras.2015.10.002
G. Nangmenyi, X. Li, S. Mehrabi, E. Mintz, J. Economy, Silver-modified iron oxide nanoparticle impregnated fiberglass for disinfection of bacteria and viruses in water. Mater. Lett. 65, 1191–1193 (2011)
A.A.H. El-Bassuony, Influence of high annealing temperature on structural, magnetic and antimicrobial activity of silver chromite nanoparticles for biomedical applications. J. Inorg. Organomet. Polym. 30, 1821–1828 (2020). https://doi.org/10.1007/s10904-019-01306-w
M.J. Iqbal, Z. Ahmad, Y. Melikhov, I.C. Nlebedim, J. Magn. Magn. Mater. 324, 1088–1094 (2012)
M.H. Maklad, N.M. Shash, H.K. Abdelsalam, Structural and magnetic properties of nano grained Ni0.7−yZn0.3CayFe2O4 spinels. Eur. Phys. J. Appl. Phys. 66, 30402 (2014). https://doi.org/10.1051/epjap/2014130573
A.A.H. El-Bassuony, H.K. Abdelsalam, Modification of AgFeO2 by double nanometric delafossite to be suitable as energy storage in solar cell. J. Alloy Compd. 726, 1106–1118 (2017). https://doi.org/10.1016/j.jallcom.2017.08.087
J.G. Deng, X.B. Ding, W.C. Zhang, Y.X. Peng, J.H. Wang, X.P. Long, P. Li, A.S.C. Chan, Polymer 43, 2179 (2002)
A.A.H. El-Bassuony, W.M. Gamal, H.K. Abdelsalam, Influence of silver nanoferrite and nanochromite on the physical properties for high frequency and biomedical applications. JOM (2022). https://doi.org/10.1007/s11837-022-05315-y
M.A. Albalah, Y.A. Alsabah, D.E. Mustafa, Characteristics of coprecipitation synthesized cobalt nanoferrites and their potential in industrial wastewater treatment. SN Appl. Sci. 2, 804 (2020). https://doi.org/10.1007/s42452-020-2586-6
E. Ateia, A.A.H. El-Bassuony, Fascinating improvement in physical properties of Cd/Co nanoferrites using different rare-earth ions. J. Mater. Sci. 28, 11482–11490 (2017). https://doi.org/10.1007/s10854-017-6944-0
Y. Mouhib, M. Belaiche, Cobalt nano-ferrite synthesized by molten salt process: structural, morphological and magnetic studies. Appl. Phys. A 127, 613 (2021). https://doi.org/10.1007/s00339-021-04758-5
S.H. Kim, H.S. Lee, D.S. Ryu, S.J. Choi, D.S. Lee, Antibacterial activity of silver-nanoparticles against Staphylococcus aureus and Escherichia coli. J. Microb. Biotechnol. 39, 77–85 (2011)
M. Ahmed, S.T. Bishay, S.M. Salem-Gaballah, Structural characterization and magnetic properties of smart CuCd ferrite/LaSrCo manganite nanocomposites. J. Magn. Magn. Mater. 334, 96–101 (2013)
A.A.H. El-Bassuony, H.K. Abdelsalam, Enhancement of AgCrO2 by double nanometric delafossite to be applied in many technological applications. J. Mater. Sci. 29, 5401–5412 (2018). https://doi.org/10.1007/s10854-017-8506-x
D.K. Petrov, L.K. Eibaum, J.Z. Sun, C. Feild, P.R. Duncombe, Appl. Phys. Lett. 75, 995 (1999)
A. Hajalilou, L.P. Ferreira, M.E.M. Jorge, C.P. Reis, M.M. Cruz, Superparamagnetic Ag-Fe3O4 composites nanoparticles for magnetic fluid hyperthermia. J. Magn. Magn. Mater. 537, 168242 (2021)
B. Chudasama, A.K. Vala, N. Andhariya, R.V. Upadhyay, R.V. Mehta, Antifungal activity of multifunctional Fe3O4–Ag nanocolloids. J. Magn. Magn. Mater. 323, 1233–1237 (2011). https://doi.org/10.1016/j.jmmm.2010.11.012
E. Iglesias-Silva, J. Rivas, L.M. León Isidro, M.A. López-Quintela, Synthesis of silver coated magnetite nanoparticles. J. Non-Cryst. Solids 353, 829–831 (2007). https://doi.org/10.1016/j.jnoncrysol.2006.12.050
K.S. Shin, Y.K. Cho, J.-Y. Choi, K. Kim, Facile synthesis of silver-deposited silanized magnetite nanoparticles and their application for catalytic reduction of nitrophenols. Appl. Catal. A 413–414, 170–175 (2012). https://doi.org/10.1016/j.apcata.2011.11.006
A.W. Bauer, W.M. Kirby, C. Sherris, M. Turck, Antibiotic susceptibility testing by a standardized single disk method. Am. J. Clin. Pathol. 45, 493–496 (1966)
A.A.H. El-Bassuony, H.K. Abdelsalam, Tailoring the structural, magnetic and antimicrobial activity of AgCrO2 delafossite at high annealing temperature. J. Therm. Anal. Calorim. 138, 81–88 (2019). https://doi.org/10.1007/s10973-019-08207-7
M.A. Rehman, I. Yusoff, Y. Alias, Structural, morphological and magnetic investigations of CuCe0.2Fe1.8O4 graphene-supported nanocomposites. Ceram. Int. 42, 1399–1407 (2016)
B. Dutta, S. Checker, K.C. Barick, H.G. Salunke, V. Gota, P.A. Hassan, Malic acid grafted Fe3O4 nanoparticles for controlled drug delivery and efficient heating source for hyperthermia therapy. J. Alloys Compds. 883, 160950 (2021)
A.A.H. El-Bassuony, H.K. Abdelsalam, Correlation of heat treatment and the impurities accompanying Ag nanoparticles. Eur. Phys. J. Plus 135, 66 (2020). https://doi.org/10.1140/epjp/s13360-019-00025-y
D.B. Pawar, S.M. Rathod, Synthesis, characterization and magnetic properties of Ce3+ substituted Cu nanoferrite by sol-gel autocombustion technique. Int. J. Innov. Res. Sci. Eng. Technol. 4, 7556–7561 (2015). https://doi.org/10.15680/IJIRSET.2015.0408082
P. Scherrer, Bestimmung der Grösse und der inneren Struktur von Kolloidteilchen mittels Röntgenstrahlen. Nachr. Ges. Wiss. Göttingen 26, 98 (1918)
J.I. Langford, A.J.C. Wilson, Scherrer after sixty years: a survey and some new results in the determination of crystallite size. J. Appl. Cryst. 11, 102 (1978)
V. Uvarov, I. Popov, Metrological characterization of X-ray diffraction methods for determination of crystallite size in nanoscale materials. Mater. Charact. 85, 111 (2013)
R.D. Waldron, Infrared spectra of ferrites. Phys. Rev. 99, 1727–1735 (1955)
A.A.H. El-Bassuony, H.K. Abdelsalam, Impacts of hematite, bunsenite and maghemite impurities on the physical and antimicrobial properties of silver nanoparticles. Eur. Phys. J. Plus 135, 64 (2020). https://doi.org/10.1140/epjp/s13360-020-00139-8
T. Caronna, F. Fontana, I.N. Sora, R. Pelosato, L. Viganò, Solid State Ionics 181, 1355–1358 (2010)
S.A. Mazen, S.F. Mansour, E. Dhahri, H.M. Zaki, T.A. Elmosalami, The infrared absorption and dielectric properties of Li-Ga ferrite. J. Alloy Compd. 470, 294–300 (2009)
A.A.H. El-Bassuony, H.K. Abdelsalam, Attractive improvement in structural, magnetic, optical, and antimicrobial activity of silver delafossite by Fe/Cr doping. J. Supercond. Novel Magn. 31, 3691–3703 (2018). https://doi.org/10.1007/s10948-018-4627-6
B. Aslibeiki, Nanostructural, magnetic and electrical properties of Ag-doped Mn-ferrite nanoparticles. Curr. Appl. Phys. 14, 1659–1664 (2014)
A.A.H. El-Bassuony, H.K. Abdelsalam, Fascinating study of the physical properties of a novel nanometric delafossite for biomedical applications. JOM 71, 1866–1873 (2019). https://doi.org/10.1007/s11837-019-03415-w
M. Amami, F. Jlaiel, P. Strobel, A. BenSalah, Structural, magnetic and electric properties of Delafossite-type oxide, Cu1-xAgxCrO2 (0 < x < 0.5). IOP Ser. Mater. Sci. Eng. 13, 012001 (2010)
A.A.H. El-Bassuony, Tuning the structural and magnetic properties on Cu/Cr nanoferrite using different rare-earth ions. J. Mater. Sci. 29, 3259–3269 (2017). https://doi.org/10.1007/s10854-017-8261-z
A. Manohar, V. Vijayakanth, R. Hong, Solvothermal reflux synthesis of NiFe2O4 nanocrystals dielectric and magnetic hyperthermia properties. J. Mater. Sci. 31, 799–806 (2020). https://doi.org/10.1007/s10854-019-02588-z
M.H. Maklad, N.M. Shash, H.K. Abdelsalam, Synthesis, characterization and magnetic properties of nanocrystalline Ni1−xZnxFe2O4 spinels via coprecipitation precursor. Int. J. Mod. Phys. B 28, 1450165 (2014). https://doi.org/10.1142/S0217979214501653
A. Manohar, C. Krishnamoorthi, Structural, optical, dielectric and magnetic properties of CaFe2O4 nanocrystals prepared by solvothermal reflux method. J. Alloy Compd. 722, 818–827 (2017)
A.A.H. El-Bassuony, H.K. Abdelsalam, Giant exchange bias of hysteresis loops on Cr3+-doped Ag nanoparticles. J. Supercond. Nov. Magn. 31, 1539–1544 (2018). https://doi.org/10.1007/s10948-017-4340-x
M.N. Akhtar, M. Saleem, M.A. Khan, Al-doped spinel and garnet nanostructured ferrites for microwave frequency C and X-band applications. J. Phys. Chem. Solids 123, 260–265 (2018)
P. Akhtar, M.N. Akhtar, M.A. Baqir, A. Ahmad, M.U. Khallidoon, M. Farhan, M.A. Khan, Structural and magnetic evaluations of rare-earths (Tb, Pr, Ce, Gd, Y)-doped spinel ferrites for high frequency and switching applications. J. Mater. Sci. 32, 7692–7703 (2021)
R. Gieré, Magnetite in the human body: biogenic vs. anthropogenic. PNAS 113, 11986–11987 (2016)
M.A. Sayed, A.A.H.H.K. El-BassuonyAbdelsalam, Evaluation of antimicrobial properties of a Novel synthesized nanometric delafossite. Braz. J. Microbiol. 51, 1475–1482 (2020). https://doi.org/10.1007/s42770-020-00366-2
W.M. Gamal, A.A.H. El-Bassuony, H.K. Abdelsalam, S.M. Abd El Wahab, Role of elastic and optical properties on silver nanoferrite and nanochromite for optical switch device applications. J. Mater. Sci. 32, 21590–21602 (2021). https://doi.org/10.1007/s10854-021-06667-y
A.A.H. El-Bassuony, H.K. Abdelsalam, Synthesis, characterization, magnetic and antimicrobial properties of silver chromite nanoparticles. J. Mater. Sci. 31, 3662–3673 (2020). https://doi.org/10.1007/s10854-020-02924-8
M.A. Sayed, T.M.A.A. El-Rahman, H.K. Abdelsalam, et al. Attractive study of the antimicrobial, antiviral, and cytotoxic activity of novel synthesized silver chromite nanocomposites. BMC Chem. 16, 39 (2022). https://doi.org/10.1186/s13065-022-00832-y
Funding
Open access funding provided by The Science, Technology & Innovation Funding Authority (STDF) in cooperation with The Egyptian Knowledge Bank (EKB). The authors have not disclosed any funding.
Author information
Authors and Affiliations
Contributions
AAHE-B, WMG, HKA: Methodology, writing original draft preparation, investigation, Data Curation.
Corresponding author
Ethics declarations
Conflict of interest
The authors declare that they have no conflict of interest.
Additional information
Publisher's Note
Springer Nature remains neutral with regard to jurisdictional claims in published maps and institutional affiliations.
Rights and permissions
Open Access This article is licensed under a Creative Commons Attribution 4.0 International License, which permits use, sharing, adaptation, distribution and reproduction in any medium or format, as long as you give appropriate credit to the original author(s) and the source, provide a link to the Creative Commons licence, and indicate if changes were made. The images or other third party material in this article are included in the article's Creative Commons licence, unless indicated otherwise in a credit line to the material. If material is not included in the article's Creative Commons licence and your intended use is not permitted by statutory regulation or exceeds the permitted use, you will need to obtain permission directly from the copyright holder. To view a copy of this licence, visit http://creativecommons.org/licenses/by/4.0/.
About this article
Cite this article
El-Bassuony, A.A.H., Gamal, W.M. & Abdelsalam, H.K. Fascinating study of adding nanocomposite cobalt nano ferrite to silver nanoparticles accompanied magnetite impurity. J Mater Sci: Mater Electron 33, 16219–16235 (2022). https://doi.org/10.1007/s10854-022-08516-y
Received:
Accepted:
Published:
Issue Date:
DOI: https://doi.org/10.1007/s10854-022-08516-y