Abstract
In this work, aluminium scandium nitride (\(\hbox {Al}_{1-x}\hbox {Sc}_{x}\hbox {N}\)) thin films are deposited by reactive DC magnetron co-sputtering on Pt(111) layers. The sputtering power of the Sc target is varied in a broad range up to 900 W to effectively vary the Sc content later assessed using energy-dispersive X-ray spectroscopy (EDX). We show that commonly used X-ray diffraction techniques may yield ambiguous results, and thus, additional verification is crucial to substantiate the actual composition of the sputtered thin films. Therefore, we employ optical spectroscopy techniques, such as Raman and Fourier transformed infra-red (FTIR) spectroscopies in order to study the vibrational properties of the alloys. Our Raman spectra show that low Sc content leads to a phase separation with the formation of cubic ScN and AlScN phases during the sputtering process. Nevertheless, small amounts of the wurtzite phase are detected in the rock-salt dominated samples owing to the enhancement of the \(\hbox {A}_{{1}}\)(LO) phonon mode by the Berreman effect in the FTIR spectra. The Sc-induced shifts of the AlN phonon modes are determined in a broad range of Sc content.
Similar content being viewed by others
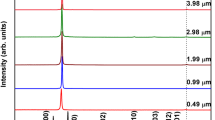
Avoid common mistakes on your manuscript.
Introduction
The alloys of aluminium nitride (AlN) with group III atoms, such as Scandium and Yttrium, are known to exhibit improved piezoelectric performance in comparison with pure AlN. The formation of purely wurtzite alloys remains a challenging task due to the complex phase diagram with pure wurtzite and rock-salt (ScN) phases at the extremities. Aluminium nitride, a III-V wide-band gap semiconductor, is successfully utilized as a piezoactive layer in various modern micro-electro-mechanical systems [1, 2]. Such prevalence of AlN-based devices stems from the possibility of the low-temperature wafer-scale synthesis of AlN, its compatibility with CMOS technology, and environmental considerations [3, 4]. Nevertheless, the quality of the piezoelectric performance of AlN is rather low [5,6,7] in comparison with prominent piezoelectric materials, such as lead zirconate titanate [8], and slightly lower than another tetrahedrally bonded semiconductor, ZnO [9]. This inherently limits its broader application. One common solution for the improvement of piezoelectric coefficients of AlN is alloying with Sc atoms [10, 11], which was shown to increase the \(d_{33}\) piezoelectric constant up to 400\(\%\) in the case of 43\(\%\) Sc content in the ternary AlScN compound [12]. This effect was shown to be linked to the modification of the wurtzite matrix by Sc atoms, which results in the softening of the structure manifested by the decrease in the stiffness constants and the increase in the internal strain [13, 14]. The precise Sc incorporation, however, remains challenging due to a complex phase diagram, where a simultaneous formation of wurtzite, hexagonal, and cubic phases of \(\hbox {Al}_{x-1}\hbox {Sc}_{{x}}\hbox {N}\) as well as its parental phases cannot be fully avoided [12, 15]. Provided that the growth method of choice for industrial applications is reactive magnetron sputtering (RMS), an accurate control over the phase formation is impeded by the stochastic nature of this method. Nevertheless, it was successfully demonstrated that RMS is capable to produce AlScN thin films of a considerable crystalline quality [10, 16]. The influence of growth conditions and underlying substrates on the formation of AlScN utilizing tilted targets of Al and Sc, which are co-sputtered with pure \(\hbox {N}_{{2}}\) gas under vacuum conditions of about 1 Pa, was investigated. [17, 18]. The quality of AlScN films can be further improved by normal-incidence sputtering of a single target with an Al-Sc alloy [11, 19, 20], which, however, limits the variation of the compositional ratio between the metal atoms.
Due to the intricate phase diagram of the AlScN alloy, where AlN is predominantly wurtzite on one end, whereas ScN is predominantly cubic on the other one, careful determination of the intermediate stages must be performed. At present, such samples are routinely studied by X-ray diffraction (XRD) [10, 16, 21], which mainly provides information on the phase composition, the orientation, and the size of AlScN grains. Yet, the success of this method is prone to large volumes of the crystallites probed, and thus, it is not suitable for very thin films or layers within the films. Moreover, it is difficult to distinguish between the alloy broadening, which is typical for such crystals, and the structural disorder in the samples by analysing XRD data. An alternative way to investigate the structural quality of AlScN films is to employ optical methods, such as Raman and infra-red (IR) spectroscopies. Both techniques provide the phonon properties of crystals, which allow the geometric and compositional changes upon alloying to be tracked with high sensitivity and measurement speed, being essential for the industrial manufacturing of AlScN. Moreover, the optical methods have negligible limitations towards the crystallite size or film thicknesses, making them extremely suitable for studies of binary and ternary III–V compounds [22].
However, the measured spectra cannot be approached in a straightforward manner as in the case of X-ray diffractograms, directly extracting the orientation and inter-planar distances of a crystal without a prior calibration. Deng et al. [23] showed the relation between the positions of the spectral bands and the Sc content, demonstrating that the Sc alloying of AlN leads to the softening of its vibrational modes as a result of an increased ionicity, leading to the decrease in bond angles and their extension. In the range of the Sc content investigated (up to 16\(\%\)), the alloy is supposed to retain the wurtzite structure since the formation of the other phases, mainly, the cubic one, is expected at higher Sc content (around 40\(\%\)). However, the vanishing of the spectral band of wurtzite AlN (\(\hbox {E}_2\)(high)) can be seen in the Raman spectra already at 10\(\%\) of Sc. This suggests that either no wurtzite phase is formed at such low Sc content or the sensitivity of the experimental set-up was not sufficient for its detection. The latter can be improved using an excitation wavelength closer to the optical absorption edge, which, in the case of AlScN, lies in the UV range (ca. 6 eV) [24].
In this work, we study the dependence of the Sc content related to the sputtering power of the corresponding target on the vibrational properties of \(\hbox {Al}_{1-x}\hbox {Sc}_{x}\hbox {N}\) thin films. We show that the optical spectroscopy results can be used to detect various crystalline phases formed during the co-sputtering procedure surpassing the sensitivity of the XRD results. In particular, we observe the formation of the wurtzite phase in the case of the highest Sc content via the presence of the \(\hbox {A}_{{1}}\)(LO) mode in the IR spectrum because of the Berreman enhancement mechanism. Combining the evidence from the methods used, we conclude that the \(\hbox {Al}_{1-x}\hbox {Sc}_{x}\hbox {N}\) films grown with high Sc content relative to Al exhibit the presence of wurtzite-like and cubic-like alloys. The compositional disorder seems to remain a common outcome of the magnetron sputtering of \(\hbox {Al}_{1-x}\hbox {Sc}_{x}\hbox {N}\) films.
Experimental details
The \(\hbox {Al}_{1-x}\hbox {Sc}_{x}\hbox {N}\) thin films were prepared utilizing reactive magnetron sputtering from pure Al and Sc targets with a size of 236 mm using a MS150x4 AE-B set-up (FHR Anlagenbau GmbH, Germany). (100)-oriented Si wafers of 150 mm in diameter with 500 nm \(\hbox {SiO}_{{2}}\) and 100 nm \(\hbox {Si}_3\hbox {N}_4\) layers were used as substrates onto which an underlying thin Pt film of 100 nm on a buffer of 20 nm Ti was deposited for all samples completing the multi-layer stack used in the MEMS devices. During the deposition process, the substrates were heated to 350 \(^\circ\)C . The chamber was evacuated to a base pressure of \(7 \times 10^{-3}\) Pa. A pure N\({_2}\) flow of 200 sccm was introduced into the chamber as sputtering gas. During the thin film deposition, the power of the Al target was constant at 1000 W corresponding to a power density of \(0.9\hbox { W}\cdot \hbox {cm}^{-2}\), while the power of the Sc target, \(\hbox {P}_{{Sc}}\), was varied from 0 to 900 W corresponding to a variation in power density up to \(0.8\hbox { W}\cdot \hbox {cm}^{-2}\) in order to grow \(\hbox {Al}_{1-x}\hbox {Sc}_{x}\hbox {N}\) thin films with different Sc concentration. As the pulsed direct current sputtering method was used, no arcing between the charged elements was observed. The X-ray diffraction (XRD) measurements, 2\(\theta /\theta\) scans, were performed using a Rigaku Smartlab diffractometer equipped with a Ge(220) 2-bounce monochromator providing CuK\(\alpha\) radiation at a wavelength of 1.5406 Å. The scans were fitted with pseudo-Voigt functions. The energy-dispersive spectrometry of X-rays (EDX) was performed using a Scanning Electron Microscope Nova NanoSEM (FEI) with Schottky field emission gun. The measurements to determine the scandium content of the films by the aluminium and scandium K\(\alpha\)-lines were performed using an Al X-ray source at the acceleration voltage of 15 kV. It is well known that a precise assessment of the N content by EDX is difficult since the nitrogen K\(\alpha\)- and scandium L\(\alpha\)-lines are largely superimposed [17, 18]. Therefore, the estimation of the scandium concentrations was performed without consideration of nitrogen relating it to aluminium and scandium contents in atomic percent. The Raman scattering experiments were performed with a LabRam HR800 micro-Raman system at room temperature. The excitation source was a \(\lambda =\) 514.7 nm line from a Cobolt DPSS laser with a power of 0.83 mW. The laser beam was focused on the sample surface with a 100\(\times\) objective lens (NA=0.9) and then the scattering light was dispersed by a \(600\;~{ln}\cdot{mm}^{-1}\) diffraction grating with a spectral resolution of \(3.1\hbox { cm}^{-1}\). The Raman spectra were recorded with a charge-coupled device (CCD) detector in the \(z(xx){\bar{z}}\) backscattering configuration. The \(\lambda =325.0\hbox { nm}\) line of a HeCd laser with a power of 4.74 mW was also used in this study. For the latter, the light was focused using a \(40\times\) objective and a \(2400\;~{ln}\cdot{mm}^{-1}\) grating was used with a spectral resolution of \(2.3\hbox { cm}^{-1}\). Infrared reflectance spectra in the mid-IR range (\(400--6500\hbox { cm}^{-1}\)) were obtained using a Bruker Vertex 80v FTIR spectrometer with a spectral resolution of \(4\hbox { cm}^{-1}\). An angle of incidence of \(75^{\circ }\) was chosen to excite phonon modes polarized perpendicular to the film surface. The analysis of FTIR reflectance spectra was performed with the SCOUT software [25]. The thicknesses of the grown \(\hbox {Al}_{1-x}\hbox {Sc}_{x}\hbox {N}\) thin films were determined as a result of the spectral fitting showing a variation in the range of 100-300 nm. The surface morphology of the samples was obtained and analysed by an atomic force microscopy (AFM) Keysight 5500 operating in tapping mode with a tip apex radius of 10 nm. Roughness and grain size were determined using the Gwyddion software [26].
Results and discussion
The Sc content in \(\hbox {Al}_{1-x}\hbox {Sc}_{x}\hbox {N}\) can be varied with the sputtering power of the Sc target [17, 18]. Provided that the power is directly proportional to the sputtering rate, it is surmised that the amount of Sc incorporated in the films is proportional to it as well. The proportionality is demonstrated, when the fraction of Sc atoms, determined using EDX data, is plotted as a function of the sputtering power (Fig.1a). The type of relation between the Sc content and the sputtering power is revealed by fitting the experimental data with a Boltzmann sigmoid. This function commonly describes processes of continuous transitions between two states triggered by an external factor. Such transitions are especially common for physical vapour deposition techniques such as reactive co-sputtering [27, 28].
a The Sc content in the \(\hbox {Al}_{1-x}\hbox {Sc}_{x}\hbox {N}\) films plotted as a function of the sputtering power of the Sc target. The dependence is fitted with the Boltzmann sigmoid (dashed line). The error of the Sc content determination is expressed by the point size, which corresponds to \(~5\%\). b X-ray diffraction 2\(\theta\) scans for the \(\hbox {Al}_{1-x}\hbox {Sc}_{{x}}\) thin films deposited with various Sc concentrations. The diffraction profiles are plotted in a semi-logarithmic way and stacked for clarity. The lower panel contains the diffraction angles corresponding to certain planes of single crystals taken from the ICSD
Even though the Sc content in AlScN can be tuned by the power of the Sc target suggesting that Sc fully incorporates into the AlN matrix during the deposition, it may not be the only outcome of such deposition. In order to substantiate the premise of the AlScN alloying, X-ray diffraction profiles of the samples were recorded (Fig.1b). The diffractogram of the pristine AlN film (\(\hbox {P}_{Sc} = 0\hbox { W}\)) exhibits two narrow peaks around \({35.97}^{\circ }\) and \({40.06}^{\circ }\), which are attributed to the (002) plane of wurtzite AlN and the (111) plane of the Pt underlayer, respectively. The broad background between the peaks originates from their overlap with another, weaker, peak around \({37.36}^{\circ }\), which may be assigned to the diffraction from the (101) plane of wurtzite AlN as no other possible candidates were identified. The narrow peak at \({40}^{\circ }\) also shows a shoulder at lower angles with a maximum around \({39.81}^{\circ }\), which is attributed to the (110) plane of Ti, deposited as a buffer layer between \(\hbox {Si}_3\hbox {N}_4\) and Pt layers. No contributions from other underlayers (\(\hbox {SiO}_2\), \(\hbox {Si}_3\hbox {N}_4\)) are observed, which corroborates their amorphous nature. Even though highly c-axis textured AlN films are expected to form on Pt(111) [29], a misorientation of the grains occurs as a result of the geometry of the sputtering chamber, including the tilted Al target [30], which is an unavoidable condition for the co-sputtering technique, and the short distance between the target and the substrate. The latter plays a crucial role on the distribution of the sputtered ions in RMS [31]. Nevertheless, the amount of AlN grains, oriented with the c axis normal to the substrate, dominates over the tilted ones as inferred from the intensity ratio of the (002) and (101) peaks.
For the films prepared at low power supplied to the Sc target, which corresponds to Sc contents of 23–42\(\%\), the reduction of an intensity, a broadening, and a shift towards lower angles for the (002) peak are observed. However, as the power of Sc target sputtering increases further, a peak around \({36}^{\circ }\) distinctly emerges gaining in intensity and shifting towards lower angles proportionally to the Sc content in the range of 48–67\(\%\). For the highest Sc content, the diffractogram shows two clear peaks at \({35}^{\circ }\) (labelled as “I.” and “II.”), as well as another one at \({37.76}^{\circ }\) (labelled as “III.”) (Fig.1b). The peak “III.” is more intense in comparison with the one observed in the diffractogram of pure AlN and is possibly related to the formation of the abnormally oriented grains [18, 32]. The origin of the peaks at around \({35}^{\circ }\) should be scrutinized. It is characterized by a large linewidth and a clear asymmetry, originating from two contributions (“I.” and “II.”) revealed by the fitting. Hence, we can discuss them separately. The maximum of the contribution “II.” lies at a \(2\theta\) angle, which is well below the one corresponding to the (002) plane of wurtzite AlN. It is known that the substitution of Al atom by Sc atoms in AlN can result in the shift of the (002) diffraction peak towards lower angles at small Sc concentrations (< 25 \(\%\)) [10, 16, 20] as well as higher ones for the concentrations above 25 \(\%\) [18, 33] due to increasing and decreasing of the c-lattice constant, respectively. Thus, this peak cannot be assigned to the hexagonal \(\hbox {Al}_{1-x}\hbox {Sc}_{x}\hbox {N}\) alloy, since the (002)-peak should shift towards higher angles at such high (\(\gg\) 50\(\%\)) Sc content. At the same time, the peak may belong to highly strained AlN, which is often observed in sputtered films. Despite the fact that it seems unlikely to observe pure AlN, when a large number of Sc atoms is simultaneously co-sputtered, we shall not exclude the possibility of a phase separation. The contribution “I.” could be related to the (111) plane of cubic ScN (ca. \({34.5}^{\circ }\) [34, 35]) if it is under large tensile strain. Such an interpretation seems more likely since the \(\hbox {Al}_{1-x}\hbox {Sc}_{x}\hbox {N}\) alloy with a Sc content \(\ge\)50\(\%\) predominantly forms in the rock-salt crystal structure [15], therefore, rs-ScN, if separated, may be well primed by the already-formed rock-salt alloy. The formation of cubic AlN might be also considered being primed by the ScN domains, however, since it requires relatively high formation temperatures (\(\ge\)1000\(^{\circ }\)C), the probability of its formation is rather low. Thus, the sample with the highest Sc content may contain two cubic phases: rs-ScN and rs-\(\hbox {Al}_{1-x}\hbox {Sc}_{x}\hbox {N}\) alloy. Such growth process, which leads to multi-phase formation, is also supported by the analysis of the surface morphology obtained from the AFM measurements showing an average grain size in the order of 30 nm (cf. Supplemental Information). Despite the likelihood of the interpretation, while being based solely on the XRD results, it is ambiguous owing to small sizes of grains and the poor texturing [36].
Raman spectra recorded from \(\hbox {Al}_{1-x}\hbox {Sc}_{x}\hbox {N}\) thin films, deposited with varying sputtering power of the Sc target 0-900 W. The spectra were recorded with excitation wavelengths (a) \(\lambda = 514.7\) nm and (b) \(\lambda = 325.0\) nm. Both plots show spectra normalized to their maximum intensity
Figure 2 shows a set of Raman spectra recorded from the \(\hbox {Al}_{1-x}\hbox {Sc}_{x}\hbox {N}\) films grown with varied Sc content. The spectrum of pure AlN shows a strong narrow peak at \(654.9\hbox { cm}^{-1}\) and a weak broad peak centred around \(880\hbox { cm}^{-1}\) (Fig. 2a). These Raman bands can be assigned to \(\hbox {E}_{{2}}\)(high) and \(\hbox {A}_{{1}}\)(LO) phonon modes, respectively [22, 37, 38]. In general, the AlN crystal may exhibit up to six Raman bands, related to six zone-centre optical phonon modes (\(\hbox {A}_{{1}}\)(TO), \(\hbox {A}_{{1}}\)(LO), \(\hbox {E}_{{1}}\)(TO), \(\hbox {E}_{{1}}\)(LO) and 2 \(\hbox {E}_{{2}}\) modes) depending on the scattering configuration [22]. Such large number of optical phonons reflects the anisotropy of the wurtzite (point group: \(C_{6v}\)) polar crystal, for which splitting of the phonon modes along the ordinary and extraordinary optical axis as well as splitting between transverse and longitudinal modes are expected [39, 40]. In the present configuration, only \(\hbox {E}_{{2}}\)- and \(\hbox {A}_{{1}}\)-symmetry modes are observable accounting for the highly oriented c-axis growth of AlN films. The absence of the \(\hbox {E}_{{2}}\)(low) mode in the Raman spectrum is explained by the low thickness of the film, while the weak \(\hbox {A}_{{1}}\)(LO) mode corroborates the dominant orientation of the crystal grains along the c-axis.
As the power of Sc sputtering is increased yielding a Sc content of 23\(\%\), a redshift and broadening of the \(\hbox {E}_{{2}}\)(high) phonon mode are observed. The further increase in the Sc amount results in the vanishing of the wurtzite-related Raman bands. This is interpreted as the deterioration of wz-structure in the \(\hbox {Al}_{1-x}\hbox {Sc}_{x}\hbox {N}\) solid solution, which agrees with the XRD results. The Raman spectra of the samples with a high Sc content (> 40\(\%\)) exhibit one major broad band centred around \(715\hbox { cm}^{-1}\). This band persistently appears in the spectra of the samples deposited with a high Sc power. Also a weaker band around \(1010\hbox { cm}^{-1}\) gains in intensity proportionally to the Sc content. The frequency of the \(\hbox {E}_{{2}}\)(high) band in the pristine AlN thin film is lower by \(2.5\hbox { cm}^{-1}\) than the value of bulk material, \(657.4\hbox { cm}^{-1}\), reported by Davydov et al. [41]. The frequency difference is attributed to the internal tensile strain naturally appearing in thin films prepared by reactive magnetron sputtering [38, 42]. The inverse relation between the phonon lifetime and the fullwidth at half maximum (FWHM) of a corresponding Raman peak allows the crystal quality of thin films to be assessed [43]. The FWHM of the \(\hbox {E}_{{2}}\)(high) band is \(22.4\hbox { cm}^{-1}\), which is larger than the one of a high quality AlN bulk crystal, which was reported by Kuball et al. [44] to be around \(3\hbox { cm}^{-1}\) or even the one of AlN films produced by magnetron sputtering [45]. This corroborates the high level of defect density in the deposited thin films. With increase in Sc content, the \(\hbox {E}_{{2}}\)(high) mode shifts from 654.9 to \(647.6\hbox { cm}^{-1}\) and the FWHM increases from 22.4 to \(44.0\hbox { cm}^{-1}\), indicating the bond softening and increasing disorder in the wurtzite \(\hbox {Al}_{1-x}\hbox {Sc}_{x}\hbox {N}\) lattice due to the incorporation of Sc atoms as reported by Deng et al. [23]. The broad spectral feature around \(715\hbox { cm}^{-1}\), observed in the spectra of the samples with a Sc content \(>~25\%\), could be associated with the contribution of the rock-salt structure in the alloys [46,47,48,49,50]. One-phonon bands are Raman-inactive in a crystal with the rock-salt structure because of symmetry considerations [51]. Thus, this broad band can be attributed to multiple-phonon Raman scattering such as (i) second-order phonon scattering or (\(ii\)) defect-induced phonon density of states (pDOS) [46]. According to the density of states of rock-salt AlN reported by Manjón et al. [46], the broad band at \(715\hbox { cm}^{-1}\) can combine the contribution of TA+TO(L) phonon modes near \(700\hbox { cm}^{-1}\), and the one from TA+TO(\(\Gamma\)-K-X-L) modes at approximately \(760\hbox { cm}^{-1}\). On the other hand, the high density of defects can relax the Raman selection rules and thus the pDOS of rs-AlN or rs-ScN can also be observed. According to the pDOS of rs-AlN, a high density of states is located around \(700\hbox { cm}^{-1}\), which is associated with the LO(X) phonon mode [46, 47], being close to the frequency observed. From the theoretical studies of rs-ScN by Paudel et al. [48], the pDOS shows a high density of states at about \(657\hbox { cm}^{-1}\), which corresponds to the LO(L) phonon mode. Such mode of rs-ScN was experimentally reported at \(677\hbox { cm}^{-1}\) [48,49,50]. One can see that the phonon modes of the rock-salt compounds are found in the spectral range of 657-\(760\hbox { cm}^{-1}\). Therefore, we cannot unambiguously distinguish between rs-AlN and rs-ScN phases as well as the alloy of rs-\(\hbox {Al}_{1-x}\hbox {Sc}_{x}\hbox {N}\); however, it is clear that the very broad band centred around \(715\hbox { cm}^{-1}\) belongs to one of these cubic phases or their combination.
In order to exclude the possibility to assign the observed modes to species, which are unrelated to AlN or \(\hbox {Al}_{1-x}\hbox {Sc}_{x}\hbox {N}\), we recorded Raman spectra using an UV excitation source, which improves the Raman intensity of the AlN bands approaching the conditions for the resonance Raman scattering. Additionally, the short-wavelength excitation also has a lower penetration depth as the absorption coefficient commences to increase with higher energies. Such situation is beneficial for the investigation of thin films since a larger amount of scattered light originates from the film itself and thus the spectrum is less influenced by the light scattered of the substrate. The penetration depth \(\delta ({\lambda })\) for a semiconductor is approximated by \(\delta ({\lambda })=1/\alpha ({\lambda })\), where \(\alpha\) is the absorption coefficient. Due to the back-scattering geometry, the light propagates inside a thin film twice: along the incident and scattered channels. Hence, the equation, which describes the information depth is \(\delta \approx 1/(2\alpha )\) [52, 53]. Using this equation and the values of \(\alpha\) for AlN, reported by Zarwasch et al. [54], we estimate the penetration depths for the laserlines used for our measurements: \(\delta (514.7)\approx\)190 nm and \(\delta (325.0)\approx\)7 nm.
Figure 2b shows the Raman spectra of the samples recorded using the \(\lambda\)=325 nm laserline. The \(\hbox {E}_{{2}}\)(high) and \(\hbox {A}_{{1}}\)(LO) modes for pristine wz-AlN are detected at about \(654.5\hbox { cm}^{-1}\) and \(880.3\hbox { cm}^{-1}\). The mode positions are found in a very good agreement with the ones recorded using \(\lambda _{\mathrm{exc}}=514.7\hbox { nm}\) (within an error bar of \(0.4\hbox { cm}^{-1}\)), which indicates the out-of-plane homogeneity of the thin film. The FWHM of the \(\hbox {E}_{{2}}\)(high) band is \(20.3\hbox { cm}^{-1}\). The Raman peak of the \(\hbox {A}_{{1}}\)(LO) mode has a more pronounced appearance. Both the \(\hbox {A}_{{1}}\)(LO) band and the narrow peak of the \(\hbox {E}_{{2}}\)(high) mode suggest a smaller defect density close to the surface of the thin film in comparison with the average one obtained with longer excitation wavelength. The trends in Raman spectra with increase in Sc content are also reproduced. The \(\hbox {E}_{{2}}\)(high) mode can be followed only at very low Sc concentrations. Starting from the sample with a Sc content of \(42\%\), which corresponds to \(\hbox {P}_{{Sc}}=400\hbox { W}\), only broad spectral features located in the range of 600-\(900\hbox { cm}^{-1}\) are seen. We treat these bands as a whole since their fine structure is obscure due to low signals and thus a visible impact of the detector sensitivity. The latter is indicated by the jagged spectral profile. The linewidths of these broad bands in Fig. 2b are in the range of 140-\(180\hbox { cm}^{-1}\), which is broader than the ones observed with \({\lambda }_{exc}=514.7\hbox { nm}\). This can indicate that there is an additional mechanism that decreases the phonon lifetime, in particular, free charge carriers excited with the \(\lambda _{\mathrm{exc}}\)=325 nm laserline and trapped at oxygen vacancies [55].
By comparing the spectra recorded using two different excitation energies, the peak marked with asterisk at \(819.6\hbox { cm}^{-1}\) and the peak at \(1015\hbox { cm}^{-1}\) (Figure 2a) can be assigned. The \(\hbox {Al}_{1-x}\hbox {Sc}_{{x}}\) thin films were grown with a thickness in the range of 100-200 nm. Taking into account the penetration depth of the \(\lambda =514.7\hbox { nm}\) laserline (\(\delta _{\alpha }(514.7)\approx \ 190\hbox { nm}\)), these bands could originate from the underlying Pt layer, and thus, one possible assignment for these peaks is \(\hbox {PtN}_{{2}}\) [56].
The observed redshift and the broadening of the \(\hbox {E}_{{2}}\)(high) band due to the incorporation of Sc atoms, clearly indicate the deterioration of the wurtzite \(\hbox {Al}_{1-x}\hbox {Sc}_{x}\hbox {N}\) phase. However, its dependence on Sc content cannot be followed at high Sc content values. This might be interpreted as the absence of the wurtzite phase for x \(\ge\) 0.25. In fact, the rock-salt phase should dominate in a solid solution only for x \(\ge\) 0.5, in accordance with the calculated mixing enthalpy in this ternary alloy reported by Höglund et al. [15]. Yet, the amount of rock-salt structures seems to dominate at low Sc concentration, when judged from Raman data only. Nevertheless, the Raman results confirm previous reports, allowing only qualitative analysis in the case of Sc-rich \(\hbox {Al}_{1-x}\hbox {Sc}_{x}\hbox {N}\) alloys.
a Stacked FTIR reflectance spectra of the \(\hbox {Al}_{1-x}\hbox {Sc}_{x}\hbox {N}\) thin films grown with increased sputtering power of the Sc target. Inset: a spectrum for pure wz-AlN in the range of 500-\(750\hbox { cm}^{-1}\). The fitted curves are plotted with dashed-dotted lines. b Schematic diagram of the layer stack for a deposited sample. c The position of the phonon modes of AlScN as a function of the Sc content, determined by the spectral fitting. The error bars represent the uncertainty of the fit
Figure 3a shows the FTIR reflectance spectra of the \(\hbox {Al}_{1-x}\hbox {Sc}_{x}\hbox {N}\) samples deposited with varied Sc content recorded at room temperature. The IR spectrum of pure AlN exhibits 3 bands at \(611.1\hbox { cm}^{-1}\), \(665.0\hbox { cm}^{-1}\), and \(889.3\hbox { cm}^{-1}\), which correspond to \(\hbox {A}_{{1}}\)(TO), \(\hbox {E}_{{1}}\)(TO), and \(\hbox {A}_{{1}}\)(LO) phonon modes of wz-AlN, respectively. The frequencies are shifted in comparison with the bulk values of \(611.0\hbox { cm}^{-1}\), \(670.8\hbox { cm}^{-1}\), and \(890.0\hbox { cm}^{-1}\) [41]. This mismatch is attributed to biaxial strain in the AlN thin film [57], verifying the XRD and Raman results.
The bands related to the transverse modes exhibit an intensity much lower than the one of the longitudinal phonon (Fig. 3a, inset). The strong intensity of the \(\hbox {A}_{{1}}\)(LO) band is caused by the Berreman effect (BE) [58]. The BE can be observed in an ultra-thin film deposited on a metallic substrate having a thickness much less than the wavelength of the incident light. The surface charges in metal excited by the normal component of electric field generate a resonance at longitudinal optical mode frequencies [59]. It corresponds to the frequency at which the real part of the dielectric function of a crystal approaches zero [60, 61], which corresponds to the LO modes in a polar crystal. In the case of AlN films with high c-axis orientation, the coupling with the metallic substrate via the Berreman effect concerns the \(\hbox {A}_{{1}}\)(LO) mode only [62]. At the oblique incidence of unpolarized light, both TO and LO modes are detected simultaneously since the electric field of unpolarized light has components, which are parallel and perpendicular to the surface of the film. On the other hand, the proximity of the AlN film to the Pt layer has the side effect of suppressing the TO modes because the electric field within a film is strongly weakened [59].
With an increase in the Sc content, the \(\hbox {A}_{{1}}\)(LO) mode clearly exhibits a redshift and an intensity loss. For the Sc content of \(67\%\), the position of the mode reaches ca. \(790\hbox { cm}^{-1}\), being softened by over \(100\hbox { cm}^{-1}\) with respect to the case of pure AlN. Similar trends can be observed for the transverse phonon modes as well, but, due of their low intensity, their tracking is complicated. We explain this behaviour by the Al-N bond softening and a reduction in the crystalline quality of the films as a result of the incorporation of Sc atoms. The latter is corroborated by the broadening of this mode, which becomes broader as more Sc atoms are alloyed into the AlN lattice.
Generally, the simulation of the optical properties of layer stacks is mandatory for the analysis of FTIR spectra since the interplay between mode parameters, layer thicknesses, and refractive indices of the layers affects positions and shapes of IR bands. The samples are considered as a stack of 4 layers, which consists of (i) a thin film layer of deposited \(\hbox {Al}_{1-x}\hbox {Sc}_{x}\hbox {N}\) alloy with thickness d; (ii) a 100 nm Pt layer; (iii) a 500 nm silicon dioxide film; (iv) and a semi-infinite layer of the Si substrate. In order to describe the scattering losses at the surface, we also applied a roughness layer on top of the \(\hbox {Al}_{1-x}\hbox {Sc}_{x}\hbox {N}\) layer. The complete layer structure is shown in Figure 3b. The \(\hbox {A}_{{1}}\) and \(\hbox {E}_{{1}}\) transverse optical phonon modes of \(\hbox {Al}_{1-x}\hbox {Sc}_{x}\hbox {N}\) were modelled using the Kim oscillator function, which is an extension of the harmonic oscillator model with a flexible broadening parameter [63]. The choice of this model is motivated by the consistency in the fitting of the results of the vibrational spectroscopy measurements. The complex dielectric function is given by:
with
The parameter \(\sigma _{k}\ge 0\) is the so-called Gauss–Lorentz-switch and used to introduce the Gaussian broadening in addition to the Lorentzian one coupled to the damping factor \(\gamma _{k}(\omega )\) [64]. The position of LO modes depends on the resonance frequency of oscillators and can be described with the energy loss function,
The simulated spectra nearly perfectly overlap with the experimental data (Fig. 3a). This allows not only an accurate determination of the position of the LO mode but also the determination of the transverse phonon frequencies. The latter directly comprises the dielectric function model. Thus, the phonon frequencies are plotted as a function of the Sc content (Fig.3c). Linear negative slopes are found and fitted for each mode:
Up to a Sc content of 58\(\%\), the modes linearly shift towards lower energies. When the Sc concentration is above 58\(\%\), the intensity of the transverse modes dramatically drops. This introduces a considerable uncertainty in the determination of their positions and hence they should not be trusted. However, the trend of the longitudinal mode remains linear even at high concentrations of Sc. This indicates the presence of the wurtzite phase in seemingly rock-salt dominating part of the \(\hbox {Al}_{1-x}\hbox {Sc}_{x}\hbox {N}\) phase diagram [15]. However, the size of the crystallites with wurtzite structure is small and thus their presence cannot be deduced from the XRD results. At the same time, the crystallites with the rock-salt structure must also be considerably small and show no preferable orientation, as judged from the XRD results and confirmed by the absence of the phonon mode of ScN around \(363\hbox { cm}^{-1}\) in the far-IR region [50] (not shown). Nevertheless, the presence of the rock-salt structure cannot be debated taking into account the Raman results. Using our FTIR data, the alloying also affects the effective value of the high-frequency dielectric constant, obtained from fitting results: \(\epsilon _{\infty } = 3.45 + 2.27\cdot x\). Its direct proportionality to the Sc content indicates the semi-metallic nature of ScN [50]. Despite that the linear dependence of the high-frequency dielectric constant agrees with the previously published reports [23], a difference in the values is observed. The lower values of the optical dielectric constant stem from a low density of the films produced, being decreased by the crystalline grains of non-AlN origin as well as voids.
Conclusion
The dependence of the structural properties of \(\hbox {Al}_{1-x}\hbox {Sc}_{x}\hbox {N}\) thin films deposited on Pt(111) by reactive magnetron co-sputtering with varied power applied to the Sc target is studied. The sputtering power is proportional to the amount of deposited Sc atoms within the Boltzmann sigmoidal framework. The alloying of AlN with Sc atoms is observed via the combination of diffraction and optical spectroscopy techniques, which enabled the multiple phase co-formation to be unravelled. Both Raman spectroscopy and XRD results clearly indicate the presence of cubic \(\hbox {Al}_{1-x}\hbox {Sc}_{x}\hbox {N}\) and ScN phases for Sc contents of > 25\(\%\) but cease to detect a wurtzite phase entirely. The latter problem is circumvented by the infrared reflectance measurements, which demonstrate high sensitivity of the detection possible due to the Berreman effect, enhancing the signal in the proximity of the metallic underlayer. In particular, the red-shift of the \(\hbox {A}_{{1}}\)(LO) mode, stemming from the Sc-induced softening of the hexagonal lattice of AlN, is shown to be directly proportional to the Sc amount, and thus, it can be used for its determination in the solid \(\hbox {Al}_{1-x}\hbox {Sc}_{x}\hbox {N}\) solutions. This allows us to surmise the effective piezoelectric performance of such thin films, being strongly dependent on the amount of the crystallites with the rock-salt structure, prior to a laborious quantification of the piezoelectric tensor.
Change history
16 July 2021
A Correction to this paper has been published: https://doi.org/10.1007/s10853-021-06301-6
References
Caliendo C, Imperatori P (2003) High-frequency, high-sensitivity acoustic sensor implemented on ALN/Si substrate. Appl Phys Lett 83(8):1641–1643
Aubert T, Bardong J, Legrani O, Elmazria O, Badreddine Assouar M, Bruckner G, Talbi A (2013) In situ high-temperature characterization of AlN-based surface acoustic wave devices. J Appl Phys 114(1):014505
Stoeckel C, Billep D, Konietzka S, Zimmermann S, Otto T (2015) Wake up MEMS for Inertial Sensors. In: 2015 IEEE International Symposium on Inertial Sensors and Systems (ISISS) Proceedings, pp. 1–4. https://doi.org/10.1109/ISISS.2015.7102385
Hou Y, Zhang M, Han G, Si C, Zhao Y, Ning J, Review A (2016) aluminum nitride MEMS contour-mode resonator. J Semicond 37(10):101001. https://doi.org/10.1088/1674-4926/37/10/101001
Turner R, Fuierer P, Newnham R, Shrout T (1994) Materials for high temperature acoustic and vibration sensors: a review. Appl Acoust 41(4):299–324. https://doi.org/10.1016/0003-682X(94)90091-4
Barth S, Bartzsch H, Glöß D, Frach P, Modes T, Zywitzki O, Suchaneck G, Gerlach G (2016) Magnetron sputtering of piezoelectric AlN and AlScN thin films and their use in energy harvesting applications. Microsyst Technol 22(7):1613–1617
Fu YQ, Luo J, Nguyen NT, Walton A, Flewitt AJ, Zu XT, Li Y, McHale G, Matthews A, Iborra E et al (2017) Advances in piezoelectric thin films for acoustic biosensors, acoustofluidics and lab-on-chip applications. Progress Mater Sci 89:31–91
Lefki K, Dormans GJM (1994) Measurement of piezoelectric coefficients of ferroelectric thin films. J Appl Phys 76(3):1764–1767. https://doi.org/10.1063/1.357693
Zhao MH, Wang ZL, Mao SX (2004) Piezoelectric characterization of individual zinc oxide nanobelt probed by piezoresponse force microscope. Nano Lett 4(4):587–590. https://doi.org/10.1021/nl035198a
Wang W, Mayrhofer PM, He X, Gillinger M, Ye Z, Wang X, Bittner A, Schmid U, Luo JK (2014) High performance AlScN thin film based surface acoustic wave devices with large electromechanical coupling coefficient. Appl Phys Lett 105(13):133502. https://doi.org/10.1063/1.4896853
Lozzi A, Yen ET, Muralt P, Villanueva LG (2018) Al0.83Sc0.17N contour mode resonators with electromechanical coupling in excess of 4.5%. IEEE Trans Ultrasonics Ferroelectr Freq Control. https://doi.org/10.1109/TUFFC.2018.2882073
Akiyama M, Kamohara T, Kano K, Teshigahara A, Takeuchi Y, Kawahara N (2009) Enhancement of piezoelectric response in scandium aluminum nitride alloy thin films prepared by dual reactive cosputtering. Adv Mater 21(5):593–596
Gall D, Petrov I, Madsen LD, Sundgren JE, Greene JE (1998) Microstructure and electronic properties of the refractory semiconductor ScN grown on MgO(001) by ultra-high-vacuum reactive magnetron sputter deposition. J Vacuum Sci Technol A Vacuum Surf Films 16(4):2411–2417. https://doi.org/10.1116/1.581360
Tasnádi F, Alling B, Höglund C, Wingqvist G, Birch J, Hultman L, Abrikosov IA (2010) Origin of the anomalous piezoelectric response in wurtzite Sc1-xAlxN alloys. Phys Rev Lett 104(13):137601
Höglund C, Birch J, Alling B, Bareño J, Czigány Z, Persson POÅ, Wingqvist G, Zukauskaite A, Hultman L (2010) Wurtzite structure Sc1-xAlxN solid solution films grown by reactive magnetron sputter epitaxy: structural characterization and first-principles calculations. J Appl Phys 107(12):123515. https://doi.org/10.1063/1.3448235
Barth S, Bartzsch H, Gloess D, Frach P, Herzog T, Walter S, Heuer H (2014) Sputter deposition of stress-controlled piezoelectric AlN and AlScN films for ultrasonic and energy harvesting applications. IEEE Trans Ultrasonics Ferroelect Freq Control 61(8):1329–1334. https://doi.org/10.1109/TUFFC.2014.3040
Zywitzki O, Modes T, Barth S, Bartzsch H, Frach P (2017) Effect of scandium content on structure and piezoelectric properties of AlScN films deposited by reactive pulse magnetron sputtering. Surf Coat Technol 309:417–422
Lu Y, Reusch M, Kurz N, Ding A, Christoph T, Kirste L, Lebedev V, Žukauskaitė A (2018) Surface morphology and microstructure of pulsed DC magnetron sputtered piezoelectric AlN and AlScN thin films. Phys Status Solidi (a) 215(9):1700559. https://doi.org/10.1002/pssa.201700559
Matloub R, Artieda A, Sandu C, Milyutin E, Muralt P (2011) Electromechanical properties of Al0.9Sc0.1N thin films evaluated at 2.5 GHz film bulk acoustic resonators. Appl Phys Lett 99(9):092903. https://doi.org/10.1063/1.3629773
Matloub R, Hadad M, Mazzalai A, Chidambaram N, Moulard G, Sandu CS, Metzger T, Muralt P (2013) Piezoelectric Al1-xScxN thin films: a semiconductor compatible solution for mechanical energy harvesting and sensors. Appl Phys Lett 102(15):152903. https://doi.org/10.1063/1.4800231
Kurz N, Lu Y, Kirste L, Reusch M, Žukauskaitė A, Lebedev V, Ambacher O (2018) Temperature dependence of the pyroelectric coefficient of AlScN thin films. Phys Status Solidi (a) 215(13):1700831. https://doi.org/10.1002/pssa.201700831
Haböck U, Siegle H, Hoffmann A, Thomsen C (2003) Lattice dynamics in GaN and AlN probed with first-and second-order Raman spectroscopy. Phys Status Solidi (c) 6:1710–1731
Deng R, Jiang K, Gall D (2014) Optical phonon modes in AlxSc1-xN. J Appl Phys 115(1):013506
Deng R, Evans SR, Gall D (2013) Bandgap in \(\text{ Al}_{1-_x}\ \text{ Sc}_{{{x}}}\) N. Appl Phys Lett 102(11):112103. https://doi.org/10.1063/1.4795784
https://wtheiss.com. Accessed Apr 1 2020
Nečas D, Klapetek P (2011) Gwyddion: an Open-Source Software for SPM Data Analysis. Open Phys 10(1):181–188. https://doi.org/10.2478/s11534-011-0096-2
Reséndiz-Munoz J, Corona-Rivera MA, Fernández-Muñoz JL, Zapata-Torres M, Márquez-Herrera A, Ovando-Medina VM (2017) Mathematical model of Boltzmann’s sigmoidal equation applicable to the set-up of the RF-magnetron co-sputtering in thin films deposition of BaSrTiO3. Bulletin of Materials Science 40(5):1043–1047. https://doi.org/10.1007/s12034-017-1441-x
Reséndiz-Munoz J, Estrada-Martínez J, Meléndez-Lira MA, Zelaya-Ángel O, Medel-Juárez JDJ, Caballero-Briones F, Fernández-Munoz JL (2018) Temperature-power simultaneous effect on physical properties of BaxSr1-x TiO3 thin films deposited by RF-magnetron cosputtering for 0 \(\le\) x \(\le\) 1. Coatings 8(10):362. https://doi.org/10.3390/coatings8100362
Martin F, Muralt P, Dubois MA, Pezous A (2004) Thickness dependence of the properties of highly c-axis textured AlN thin films. J Vacuum Sci Technol A 22(2):361–365. https://doi.org/10.1116/1.1649343
Ravankhah M, Savaloni H (2017) Influence of deposition angle on the structural, morphological and optical properties of sputtered AlN thin films. Opt Int J Light Electron Opt 132:364–374. https://doi.org/10.1016/j.ijleo.2016.12.067
Chao S, Hsiao TC, Huang DR, Jeng TR (1992) Tilt-target magnetron sputter for deposition of magnetooptical disk. Japan J Appl Phys 31:426. https://doi.org/10.1143/JJAP.31.426
Mertin S, Heinz B, Rattunde O, Christmann G, Dubois MA, Nicolay S, Muralt P (2018) Piezoelectric and structural properties of c-axis textured aluminium scandium nitride thin films up to high scandium content. Surf Coat Technol 343:2–6. https://doi.org/10.1016/j.surfcoat.2018.01.046
Kurz N, Ding A, Urban DF, Lu Y, Kirste L, Feil NM, Žukauskaitė A, Ambacher O (2019) Experimental determination of the electro-acoustic properties of thin film AlScN using surface acoustic wave resonators. J Appl Phys 126(7):075106. https://doi.org/10.1063/1.5094611
Casamento J, Wright J, Chaudhuri R, Xing HG, Jena D (2019) Molecular beam epitaxial growth of scandium nitride on hexagonal SiC, GaN, and AlN. Appl Phys Lett 115(17):172101. https://doi.org/10.1063/1.5121329
Jain A, Ong SP, Hautier G, Chen W, Richards WD, Dacek S, Cholia S, Gunter D, Skinner D, Ceder G, Ka Persson (2013) The materials project: a materials genome approach to accelerating materials innovation. APL Mater 1(1):011002. https://doi.org/10.1063/1.4812323
Holder CF, Schaak RE (2019) Tutorial on powder X-ray diffraction for characterizing nanoscale materials. ACS Nano 13(7):7359–7365. https://doi.org/10.1021/acsnano.9b05157
McNeil LE, Grimsditch M, French RH (1993) Vibrational spectroscopy of aluminum nitride. J Am Ceram Soc 76(5):1132–1136
Prokofyeva T, Seon M, Vanbuskirk J, Holtz M, Nikishin S, Faleev N, Temkin H, Zollner S (2001) Vibrational properties of AlN grown on (111)-oriented silicon. Phys Rev B 63(12):125313
Arguello CA, Rousseau DL, Porto SPS (1969) First-Order Raman Effect in Wurtzite-Type Crystals. Phys Rev 181(3):1351. https://doi.org/10.1103/PhysRev.181.1351
Tsui HCL (2016) Characterisation of scandium-based III-nitride thin films. Ph.D. thesis, Imperial College London. https://doi.org/10.25560/43378
Davydov VY, Kitaev YE, Goncharuk I, Smirnov A, Graul J, Semchinova O, Uffmann D, Smirnov M, Mirgorodsky A, Evarestov R (1998) Phonon dispersion and Raman scattering in hexagonal GaN and AlN. Phys Rev B 58(19):12899–12907
Solonenko D, Schmidt C, Stoeckel C, Hiller K, Zahn DRT (2020) The Limits of the Post-Growth Optimization of AlN Thin Films Grown on Si(111) via Magnetron Sputtering. Physica status solidi (b) 257(5):1900400. https://doi.org/10.1002/pssb.201900400. URL https://onlinelibrary.wiley.com/doi/abs/10.1002/pssb.201900400. \_eprint: https://onlinelibrary.wiley.com/doi/pdf/10.1002/pssb.201900400
Di Bartolo B (2010) Optical Interactions in Solids. World Scientific Publishing Company, Singapore
Kuball M, Hayes J, Shi Y, Edgar J (2000) Phonon lifetimes in bulk AlN and their temperature dependence. Appl Phys Lett 77(13):1958–1960
Trodahl HJ, Martin F, Muralt P, Setter N (2006) Raman spectroscopy of sputtered AlN films: E2(high) biaxial strain dependence. Appl Phy Lett 89(6):061905. https://doi.org/10.1063/1.2335582
Manjón FJ, Errandonea D, Romero AH, Garro N, Serrano J, Kuball M (2008) Lattice dynamics of wurtzite and rocksalt AlN under high pressure: effect of compression on the crystal anisotropy of wurtzite-type semiconductors. Phys Rev B 77(20):205204
Keller K, Brendler E, Schmerler S, Roeder C, Heide G, Kortus J, Kroke E (2015) Spectroscopic characterization of Rocksalt-type aluminum nitride. J Phys Chem C 119(22):12581–12588
Paudel TR, Lambrecht WR (2009) Calculated phonon band structure and density of states and interpretation of the Raman spectrum in rocksalt ScN. Phys Rev B 79(8):085205
Saha B, Naik G, Drachev VP, Boltasseva A, Marinero EE, Sands TD (2013) Electronic and optical properties of ScN and (Sc, Mn) N thin films deposited by reactive DC-magnetron sputtering. J Appl Phys 114(6):063519
Travaglini G, Marabelli F, Monnier R, Kaldis E, Wachter P (1986) Electronic structure of ScN. Phys Rev B 34(6):3876–3882
Nakamoto K, Infrared and Raman Spectra of Inorganic and Coordination Compounds, Part A: Theory and Applications in Inorganic Chemistry (John Wiley & Sons, 2008). Google-Books-ID: 9t3h8pVgnyQC
Holtz M, Duncan WM, Zollner S, Liu R (2000) Visible and ultraviolet Raman scattering studies of Si1-xGex alloys. J Appl Phys 88(5):2523–2528. https://doi.org/10.1063/1.1287757
Fernandes P, Salomé P, Da Cunha A (2011) Study of polycrystalline Cu2ZnSnS4 films by Raman scattering. J Alloys Comp 509(28):7600–7606
Zarwasch R, Rille E, Pulker H (1992) Fundamental optical absorption edge of reactively direct current magnetron sputter-deposited AlN thin films. J Appl Phys 71(10):5275–5277
Barfels T, Fitting HJ, Gulans A, Jansons J, Springis M, Stolz H, Tale I, Veispals A (2001) Luminescence and electron transport properties of GaN and AlN layers. Radiat Measure 33(5):709–713. https://doi.org/10.1016/S1350-4487(01)00088-9
Yildiz A, Akinci Ü, Gülseren O, Sökmen I (2009) Characterization of platinum nitride from first-principles calculations. J Phys Conden Matter 21(48):485403
Wagner JM, Bechstedt F (2002) Properties of strained wurtzite GaN and AlN: Ab initio studies. Phys Rev B 66(11):115202
Berreman D (1963) Infrared absorption at longitudinal optic frequency in cubic crystal films. Phys Rev 130(6):2193–2198
Harbecke B, Heinz B, Grosse P (1985) Optical properties of thin films and the Berreman effect. Appl Phys A 38(4):263–267
Falsetti E, Nucara A, Shibayev PP, Salehi M, Moon J, Oh S, Brubach JB, Roy P, Ortolani M, Calvani P (2018) Infrared spectroscopy of the topological surface states of Bi 2 Se 3 by use of the Berreman effect. Phys Rev Lett 121(17):176803
Vassant S, Hugonin JP, Marquier F, Greffet JJ (2012) Berreman mode and epsilon near zero mode. Opt Exp 20(21):23971–23977
Passler NC, Razdolski I, Katzer DS, Storm DF, Caldwell JD, Wolf M, Paarmann A, Resonant Field Enhancement of Epsilon Near Zero Berreman Modes in an Ultrathin AlN Film. ACS Photonics 6(6):1365–1371 (2019)
Kim CC, Garland JW, Abad H, Raccah PM (1992) Modeling the optical dielectric function of semiconductors: extension of the critical-point parabolic-band approximation. Phys Rev B 45(20):11749–11767
Quinten M (2011) Optical properties of nanoparticle systems.Opt Prop Nanoparticle Syst Mie beyond. Wiley, New York
Acknowledgements
We acknowledge the financial support from ESF Nachwuchsforschergruppe “E-PISA”, 100310500.
Funding
Open Access funding enabled and organized by Projekt DEAL.
Author information
Authors and Affiliations
Corresponding author
Ethics declarations
Conflict of interest
The authors declare that they have no conflict of interest.
Additional information
Handling Editor: P. Nash.
Publisher's Note
Springer Nature remains neutral with regard to jurisdictional claims in published maps and institutional affiliations.
Electronic supplementary material
Below is the link to the electronic supplementary material.
Rights and permissions
Open Access This article is licensed under a Creative Commons Attribution 4.0 International License, which permits use, sharing, adaptation, distribution and reproduction in any medium or format, as long as you give appropriate credit to the original author(s) and the source, provide a link to the Creative Commons licence, and indicate if changes were made. The images or other third party material in this article are included in the article's Creative Commons licence, unless indicated otherwise in a credit line to the material. If material is not included in the article's Creative Commons licence and your intended use is not permitted by statutory regulation or exceeds the permitted use, you will need to obtain permission directly from the copyright holder. To view a copy of this licence, visit http://creativecommons.org/licenses/by/4.0/.
About this article
Cite this article
Solonenko, D., Lan, C., Schmidt, C. et al. Co-sputtering of \(\hbox {Al}_{1-x}\hbox {Sc}_{x}\hbox {N}\) thin films on Pt(111): a characterization by Raman and IR spectroscopies. J Mater Sci 55, 17061–17071 (2020). https://doi.org/10.1007/s10853-020-05244-8
Received:
Accepted:
Published:
Issue Date:
DOI: https://doi.org/10.1007/s10853-020-05244-8