Abstract
In this research, thermoeconomic analysis of a multi-effect desalination thermal vapor compression (MED-TVC) system integrated with a trigeneration system with a gas turbine prime mover is carried out. The integrated system comprises of a compressor, a combustion chamber, a gas turbine, a triple-pressure (low, medium and high pressures) heat recovery steam generator (HRSG) system, an absorption chiller cycle (ACC), and a multi-effect desalination (MED) system. Low pressure steam produced in the HRSG is used to drive absorption chiller cycle, medium pressure is used in desalination system and high pressure superheated steam is used for heating purposes. For thermodynamic and thermoeconomic analysis of the proposed integrated system, Engineering Equation Solver (EES) is used by employing mass, energy, exergy, and cost balance equations for each component of system. The results of the modeling showed that with the new design, the exergy efficiency in the base design will increase to 57.5%. In addition, thermoeconomic analysis revealed that the net power, heating, fresh water and cooling have the highest production cost, respectively.





















Similar content being viewed by others
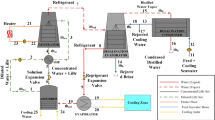
Abbreviations
- A:
-
area (m 2)
- ACC:
-
absorption chiller cycle
- AP:
-
approach point (° C)
- B:
-
brine
- c:
-
cost per exergy unit ($. (kWs)−1)
- \( \dot{C} \) :
-
cost rate ($. s −1)
- C:
-
Constant coefficient
- cc:
-
combustion chamber
- C P :
-
Specific heat capacity (kJ/kg K)
- CRF:
-
capital recovery factor
- D:
-
Distillate at desalination(kg/s)
- D r (i):
-
Distillate from the ith effect (kg/s)
- e:
-
exergy per unit of mass (kJ/kg)
- \( \dot{E} \) :
-
exergy rate (kW)
- F:
-
Total feed flow rate of MED-TVC (kg/s)
- f:
-
Feed water of desalination effects (kg/s)
- GOR:
-
Gained-Output-Ratio
- h:
-
specific enthalpy (kJ. kg −1)
- HP:
-
High Pressure (MPa)
- HRSG:
-
Heat recovery steam generator
- k:
-
interest rate
- L:
-
Latent heat \( \left( kJ.{kg}^{-1}\right) \)
- LHV:
-
Lower Heating Value \( \left( kJ.{kg}^{-1}{K}^{-1}\right) \)
- LMTD:
-
logarithmic mean temperature difference (°C)
- LP:
-
Low Pressure (MPa)
- \( \dot{m} \) :
-
mass flow rate (kg. s −1)
- MP:
-
Medium Pressure (MPa)
- MED:
-
multi-effect desalination
- MG:
-
multigeneration
- N:
-
annual number of hours (hr)
- n:
-
componets expected life
- P:
-
pressure (MPa)
- PP:
-
Pinch Point (°C)
- \( \dot{Q} \) :
-
heat transfer rate (kW)
- R:
-
reference
- r:
-
Pressure ratio \( \left(\frac{\mathrm{MPa}}{\mathrm{MPa}}\right) \)
- \( \overline{R} \) :
-
universal gases constant (J. kg −1 K −1)
- Rej:
-
Seawater reject \( \left(\frac{\mathrm{kg}}{\mathrm{s}}\right) \)
- s:
-
specific entropy (kJ. kg −1. K −1)
- T:
-
temperature (° C)
- TVC:
-
Thermal vapor compression
- \( \dot{W} \) :
-
power (kW)
- X B :
-
ammonia mass fraction of basic solution (%)
- Y:
-
molar concentration
- Z:
-
investment cost of components ($)
- \( \dot{Z} \) :
-
investment cost rate of components ($. s −1)
- η:
-
efficiency (%)
- ω:
-
humidity ratio
- ϕr :
-
maintenance factor
- λ:
-
fuel to air ratio
- ∆T:
-
Temperature difference
- a:
-
Air
- abs:
-
absorber
- cc:
-
Combustion chamber
- CH:
-
chemical
- CI:
-
capital investment
- comp:
-
compressor
- cond:
-
condenser
- D:
-
destruction
- e:
-
exit
- ec:
-
economizer
- evap:
-
evaporator
- ex:
-
exergy
- f:
-
fluid
- fuel:
-
fuel
- g:
-
gas
- gen:
-
generator
- gene:
-
generation
- heating:
-
heating
- HP:
-
High pressure
- i:
-
inlet
- is:
-
isentropic
- i:
-
ith component
- KN:
-
kinetic
- L:
-
loss
- LiBr:
-
Lithium bromide
- LP:
-
Low pressure
- mix:
-
mixing
- MP:
-
Medium pressure
- net:
-
net value
- OM:
-
operating & maintenance
- P:
-
product
- PEC:
-
initial purchase cost
- PH:
-
physical
- PT:
-
potential
- pump:
-
pump
- Q:
-
heating
- s:
-
salt
- sh:
-
superheater
- sat,HP:
-
high pressure saturation
- sat,LP:
-
Low pressure saturation
- sat,MP:
-
medium pressure saturation
- sat:
-
saturation
- sw:
-
seawater
- sol:
-
solution
- t:
-
turbine
- Ts :
-
First effect desalination inlet temperature (° C)
- tot:
-
total value
- W:
-
work
- w:
-
water
- 1, 2, …:
-
cycle locations
- 0:
-
dead state
References
Dincer I (2000) Renewable energy and sustainable development: a crucial review. Renew Sust Energ Rev 4:157–175
Dincer I, Rosen MA (1998) A worldwide perspective on energy, environment and sustainable development. Int J Energy Res 22:1305–1321
Dincer I, Rosen MA (1999) Energy, environment and sustainable development. Appl Energy 64:427–440
Hatami M, Boot MD, Ganji DD, Gorji-Bandpy M (2015a) Comparative study of different exhaust heat exchangers effect on the performance and exergy analysis of a diesel engine. Appl Therm Eng 90:23–37
Hatami M, Ganji DD, Gorji-Bandpy M (2015b) Experimental and numerical analysis of the optimized finned-tube heat exchanger for OM314 diesel exhaust exergy recovery. Energy Convers Manag 97:26–41
Ghaebi H, Amidpour M, Karimkashi S, Rezayan O (2011) Energy, exergy and thermoeconomic analysis of a combined cooling, heating and power (CCHP) system with gas turbine prime mover. Int J Energy Res 35:697–709
Ghaebi H, Saidi MH, Ahmadi P (2012) Exergoeconomic optimization of a trigeneration system for heating, cooling and power production purpose based on TRR method and using evolutionary algorithm. Appl Therm Eng 36:113–125
Orhan MF, Dincer I, Naterer GF, Rosen MA (2010) Coupling of copper–chloride hybrid thermochemical water splitting cycle with a desalination plant for hydrogen production from nuclear energy. Int J Hydrog Energy 35:1560–1574
Uche J, Serra L, Valero A (2001) Thermoeconomic optimization of a dual-purpose power and desalination plant. Desalination 136:147–158
Zamen Z, Amidpour M, Soufari SM (2009) Cost optimization of a solar humidification–dehumidification desalination unit using mathematical programming. Desalination 239:92–99
Wang Y, Lior N (2006) Performance analysis of combined humidified gas turbine power generation and multi-effect thermal vapor compression desalination systems, part 1: the desalination unit and its combination with a steam-injected gas turbine power system. Desalination 196:84–104
Alasfour FN, Darwish MA, Bin Amer AO (2005) Thermal analysis of ME-TVC+MEE desalination systems. Desalination 174:39–61
Kahraman N, Cengel YA (2005) Exergy analysis of a MSF distillation plant. Energy Convers Manag 46:2625–2636
Kafi F, Renaudin V, Alonso D, Hornut JM (2004) New MED plate desalination process: thermal performances. Desalination 166:53–62
Shih H (2005) Evaluating the technologies of thermal desalination using low-grade heat. Desalination 182:461–469
Ji J, Wang R, Li L, Ni HI (2007) Simulation and analysis of a single-effect thermal vapor compression desalination system at variable operation conditions. Chem Eng Technol 30:1633–1641
Kamali RK, Mohebinia S (2008) Experience of design and optimization of multi-effects desalination systems in Iran. Desalination 222:639–645
Kamali RK, Abbassi A, Sadough Vanini SA, Saffar Avval M (2008) Thermodynamic design and parametric study of MED-TVC. Desalination 222:596–604
Ameri M, Seif Mohammadi S, Hosseini M, Seifi M (2009) Effect of design parameters on multi-effect desalination system specifications. Desalination 245:266–283
Trostmann A (2009) Improved approach to steady state simulation of multi-effect distillation plants. Desalin Water Treat 7:93–110
Shakib SE, Amidpour M, Aghanajafi C (2012a) Simulation and optimization of multi effect desalination coupled to a gas turbine plant with HRSG consideration. Desalination 285:366–376
Shakib SE, Amidpour M, Aghanajafi C (2012b) A new approach for process optimization of a METVC desalination system. Desalin Water Treat 37:1–13
Fiorini P, Sciubba E (2005) Thermoeconomic analysis of a MSF desalination plant. Desalination 182:39–51
Sayyaadi H, Saffari A (2010) Thermoeconomic optimization of multi effect distillation desalination systems. Appl Energy 87:1122–1133
Sayyaadi H, Saffari A, Mahmoodian A (2010) Various approaches in optimization of multi effects distillation desalination systems using a hybrid meta-heuristic optimization tool. Desalination 254:138–148
Wang Y, Lior N (2007) Performance analysis of combined humidified gas turbine power generation and multi-effect thermal vapor compression desalination systems, part 2: the evaporative gas turbine based system and some discussions. Desalination 207:243–256
Nafey AS (1998) Design and simulation of sea water thermal desalination plants. Ph.D. Thesis. Leeds University, UK
Ettouney H, El-Desouky E, Al-Roumi Y (1999) Analysis of mechanical vapor compression desalination process. Int J Energy Res 23:431–451
Aly G (1983) Computer simulations of multi-effect FFE– VC systems for water desalination. Desalination 45:119–131
Aly NH, El-Fiqi AK (2003) Mechanical vapor compression desalination systems: case study. Desalination 153:143–150
El-Sayed YM (1999) Thermoeconomic of some options of large mechanical vapor mechanical vapor-compression units. Desalination 125:251–257
Bejan A, Tsatsaronis G, Moran M (1996) Thermal design and optimization. John Wiley, NewYork
Darwish MA, El-Hadik AA (1986) The multi-effect boiling desalination system and its comparison with multi-stage flash system. Desalination 60:251–265
Cengel AY, Boles AM (1994) Thermodynamics: an engineering approach. McGraw-Hill, New York
Herold KE, Radermacher R, Klein SA (1996) Absorption chillers and heat pump. CRC Press, Boca Raton
Kotas TJ (1995) The exergy method of thermal plant analysis. Krieger Publishing Company, Malabar
Kizilkan O, Sencan A, Kalogirou SA (2007) Thermoeconomic optimization of a LiBr absorption refrigeration system. Chem Eng Process 46:1376–1384
Sencan A, Yakut KA, Kalogirou SA (2005) Exergy analysis of lithium bromide/water absorption systems. Renew Energy 30:645–657
Korakiantis T, Wilson DG (1994) Methods for prediction the performance of Bryton-cycle engines. ASME J Eng Gas Turbines Power 166:381–388
Kamali RK, Abbassi A, Sadough SA (2006) A simulation model and parametric study of MED–TVC process. In: EDS International Conference, EuroMed, p 203
Bereche RP, Gonzales R, Nebra SA (2010) Exergy calculation of lithium bromide–water solution and its application in the exergetic evaluation of absorption refrigeration systems LiBr-H2O. Int J Energy Res 36:166–181
Mishra M, Das PK, Sarangi S (2004) Optimum design of cross flow plate-fin heat exchangers through genetic algorithm. Int J Heat Exch 5:379–401
Dincer I (1998) Energy and environmental impacts: present and future perspectives. Energy Sources 20:427–453
Fath SH (2000) Desalination technology. El dar Elgameia, Alexandria
Author information
Authors and Affiliations
Corresponding author
Appendices
Appendix 1: Energy balance equations for different components
Gas turbine cycle:
To calculate the compressor efficiency (η comp ), following relation can be used [39]:
where, r comp is the compressor pressure ratio. The net consumed compressor power is calculated as follows:
where, ω is the humidity ratio.
Applying energy balance equation on the combustion chamber, the input heat of the proposed integrated system can be calculated as follows:
where, λ is the fuel to air ratio.
The gas turbine isentropic efficiency can be obtained from the following relation [39]:
To calculate the output power of turbine, following relation is taken into account:
After conducting the above mentioned mathematical manipulation, the net output power of the gas turbine cycle can be calculated as follows:
HRSG:
Figure 22 illustrates the HRSG temperature profile. Pinch point is the difference between the exhaust gas temperature from the evaporator (economizer side) and the saturated liquid temperature. Triple-pressure heat recovery generator has three pinch points. The difference in the temperature of the outlet water from the economizer (T w2, T w4 and T w6) and the saturated temperature (T sat, LP , T sat, MP and T sat, HP ) is called the approach point, which its value depends on the economizer arrangement. In this study, the values of approach points (AP LP , AP MP and AP HP ) are assumed 5° C
The feed water enters to LP economizer with temperature of T w1 and is heated to T w2 by extracting heat of the flue gas. The T w2 is calculated as follows:
Similarly, T w4 and T w6 are calculated:
where, T sat, LP , T sat, MP and T sat, HP are saturated steam temperatures of low pressure, medium pressure and high pressure streams.
Similarly, from Fig. 22, we have:
The flue gas entered HRSG with the temperatures of T4, g3, T4, g5 and T4, g7 are calculated from the Eqs. (39–41):
Applying energy balance equations for the economizers of HRSG, feed water mass flow rate (or heating mass flow rate) and also T 4, g4 and T 4, g6 can be obtained as follows:
The mas flow rate of absorption chiller, desalination system and T 4, g2 are obtained from the following relations:
Now, energy balance is applied to HP superheater of the HRSG for calculating the temperature of the superheated process steam:
The amount of heating capacity produced in the system is calculated as below:
Absorption chiller cycle:
For thermodynamic analysis of the absorption chiller cycle, the energy balance equation for each component of system can be applied using Eqs. (1, 2). These employed equations are given below:
-
Evaporator:
-
Absorber:
-
Pump
-
Heat exchanger
-
Generator
-
Condenser
The amount of cooling capacity of the chiller is equal to the evaporator load:
MED-TVC desalination plant system:
MED system is divided into five sub-systems and mass and energy balances are applied for each of them as follows:
-
Steam ejector and desuperheater:
where, \( {\dot{\mathrm{m}}}_{24} \), \( {\dot{\mathrm{m}}}_{25} \) and \( {\dot{\mathrm{m}}}_{63} \) are the mass flow rates of the ejector motive steam, withdrawn vapor from nth effect by steam ejector and water consumed in desuperheater, respectively.
-
Effects 1,…,N:
In order to have a more efficient operational condition, it is assumed that the temperature difference of all effects is the same [25]:
where,
In this study, the mass flow rates of steam, brine and feed water of the ith effect are denoted by D(i), B(i) and f(i), respectively. In addition, the latent heat and the specific heat capacities of water in the ith effect are specified by L(i) and Cp(i), respectively. With these regards, the mass and energy balances for the effects of 1,…,N will be as follows [40]:
✓ Effects 1, 2 and 3:
✓ Effects 4,…,N:
The steam is only withdrawn in the Nth effect (inlet of steam ejector), so:
-
Feed water heater:
The energy balance for feed-water heater can be expressed as follows:
-
Condenser:
The mass and energy balance equations for the condenser can be written as follows:
where, \( {\dot{m}}_{57} \) , F, and Rej are the mass flow rates of condenser cooling water, feed water MED and the rejected water from desalination system, respectively. D(N) is the total rate of the exhaust steam of the Nth effect, D r (N) is the rate of the withdrawn steam from the effect N and T f and T sw are the feed water and seawater temperatures, respectively.
Gained-Output-Ratio (GOR) is another important parameter in thermal desalination plants which is defined as the ratio between the mass flow rate of produced fresh water and that of the consumed motive steam:
The MED-TVC plant duty can be obtained from the following relation:
The overall thermal efficiency of system can be defined as the ratio of output energies of the system (cooling, heating, power and fresh water) to the input energy supplied to the system:
where, LHV is the lower heating value of the fuel.
Appendix 2: Exergy relations
The chemical exergy is obtained by combining the combustion gases in accordance with Eq. (80). The fixed values in the numerator are the exergy of the elements substituted from Ref. [32].
The chemical exergy of LiBr-H2O solution can be obtained from the following relation [41]:
where, \( {\upvarepsilon}_{{\mathrm{H}}_2\mathrm{O}}=0.9\frac{\mathrm{kJ}}{\mathrm{kg}} \) and \( {\upvarepsilon}_{\mathrm{LiBr}}=101.6\frac{\mathrm{kJ}}{\mathrm{kg}} \). \( {\mathrm{a}}_{{\mathrm{H}}_2\mathrm{O}} \) and aLiBr coefficients are obtained from Ref. [41].
Also, chemical exergy of seawater can be obtained from the following equation [21]:
For one stream, we have:
The exergy destruction of each component in the proposed multigeneration system can be obtained from the following relationships.
-
For compressor:
-
For combustion chamber:
-
For turbine:
-
For HRSG:
-
For absorption chiller:
-
For desalination system:
The total exergy destruction rate of system can be expressed as follows:
Appendix 3
Table 10 is listed the cost flow rate, cost balance equations and auxiliary equations for the proposed multigeneration system.
Rights and permissions
About this article
Cite this article
Ghaebi, H., Abbaspour, G. Thermoeconomic analysis of an integrated multi-effect desalination thermal vapor compression (MED-TVC) system with a trigeneration system using triple-pressure HRSG. Heat Mass Transfer 54, 1337–1357 (2018). https://doi.org/10.1007/s00231-017-2226-x
Received:
Accepted:
Published:
Issue Date:
DOI: https://doi.org/10.1007/s00231-017-2226-x