Abstract
A study of dijet production in proton–proton collisions was performed at \(\sqrt{s}=7\mbox{~TeV}\) for jets with p T>35 GeV and |y|<4.7 using data collected with the CMS detector at the LHC in 2010. Events with at least one pair of jets are denoted as “inclusive”. Events with exactly one pair of jets are called “exclusive”. The ratio of the cross section of all pairwise combinations of jets to the exclusive dijet cross section as a function of the rapidity difference between jets |Δy| is measured for the first time up to |Δy|=9.2. The ratio of the cross section for the pair consisting of the most forward and the most backward jet from the inclusive sample to the exclusive dijet cross section is also presented. The predictions of the Monte Carlo event generators pythia6 and pythia8 agree with the measurements. In both ratios the herwig++ generator exhibits a more pronounced rise versus |Δy| than observed in the data. The BFKL-motivated generators cascade and hej+ariadne predict for these ratios a significantly stronger rise than observed.
Similar content being viewed by others
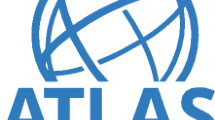

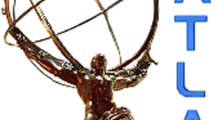
Measurement of the inclusive jet cross-section in proton-proton collisions at $$ \sqrt{s}=7 $$ TeV using 4.5 fb−1 of data with the ATLAS detector
Avoid common mistakes on your manuscript.
The measurement of inclusive jet production in pp collisions provides an important testing ground for the Standard Model. Inclusive jet production is well described at LHC energies, over a wide range in jet transverse momentum and rapidity [1, 2], by calculations at next-to-leading-order (NLO) in perturbative quantum chromodynamics (QCD) using the Dokshitzer–Gribov–Lipatov–Altarelli–Parisi (DGLAP) approach [3–7] and collinear factorization. The rapidity y is defined as y=(1/2)log[(E+p z)/(E−p z)], where E is the jet energy and p z is the component of the jet momentum along the beam axis. As shown in [8], the production of dijets with invariant mass above 165 GeV and |y| less than 2.5 is also well described by NLO predictions. When jets are well separated in rapidity, the description of the data becomes worse [2].
When the collision energy \(\sqrt{s}\) is considerably larger than the hard scattering scale given by the jet transverse momentum, p T, the average number of produced jets grows rapidly, along with the phase space available in rapidity. This kinematic regime is expected to be described by the Balitsky–Fadin–Kuraev–Lipatov (BFKL) evolution [9–11] and k T factorization [12–14]. An effective theory has been developed which describes strong interactions in this kinematic domain [15].
The ratio of the dijet production cross section in “inclusive” events to that in “exclusive” events, R incl=σ incl/σ excl, as a function of the rapidity separation |Δy| between two jets, is a sensitive probe of effects beyond collinear factorization [16]. Only jets with transverse momenta above a minimal value of \(p_{\mathrm{T}}^{\text{min}}\) are considered. Events with at least one pair of jets are denoted as “inclusive”. Events with exactly one pair of jets are called “exclusive”. In the inclusive case, the rapidity separation is evaluated for each pairwise combination of jets above threshold [16]. Mueller–Navelet jet pairs [17] are a subset of the inclusive dijet class. In this case only the jet at highest rapidity (i.e. most forward) and that at lowest rapidity (most backward) are considered. At low |Δy|, the inclusive dijet ratio, R incl, is always larger than the corresponding Mueller–Navelet dijet ratio, R MN=σ MN/σ excl, because all the jets in the rapidity interval between the Mueller–Navelet jets contribute to the inclusive cross section.
From the theoretical point of view, an advantage of the ratios R incl and R MN with respect to the individual dijet production cross sections is that the influence of the uncertainty of the parton distribution functions is greatly reduced; in addition, the ratios are particularly sensitive to the parton radiation pattern [16]. At large enough energies, the parton subprocesses involve a large number of partons with comparable transverse energies. Such subprocesses, governed by BFKL evolution, lead to an increase of the ratios with increasing |Δy| [16–18]. Experimentally, an additional advantage of the R incl and R MN ratios defined above is the cancellation of most of the systematic uncertainties affecting both numerator and denominator.
Earlier measurements of Mueller–Navelet jets with |Δy| up to 5 were made at the Tevatron by the D0 experiment [19, 20]. D0 did not find indications of BFKL effects in the azimuthal decorrelation data [19]; however, a stronger than expected \(\sqrt{s}\) dependence of the dijet production cross section was observed [20] when comparing the data at \(\sqrt{s}=630\mbox{ and }1800\text{~GeV}\) for dijets with large rapidity separation. The ATLAS Collaboration recently studied various dijet production cross section ratios at \(\sqrt{s}=7\text{~TeV}\) for |Δy|<6 [21]; the results are roughly in agreement with the DGLAP predictions. At the HERA ep collider, deviations from DGLAP were observed in studies of forward jets by the ZEUS [22, 23] and H1 [24, 25] Collaborations. However, no compelling evidence for BFKL effects was found.
The component of the CMS detector [26] most relevant for this analysis is the calorimeter system extending to pseudorapidities |η|=5.2, where η=−log[tan(θ/2)], and θ is the polar angle relative to the anticlockwise proton beam direction. The crystal electromagnetic calorimeter (ECAL) and the brass/scintillator hadronic calorimeter (HCAL) extend to pseudorapidities |η|=3.0. The HCAL cells map to an array of ECAL crystals to form calorimeter towers projecting radially outwards from the nominal interaction point. The pseudorapidity region 3.0<|η|<5.2 is covered by the hadronic forward (HF) calorimeter, which consists of steel absorber wedges with embedded radiation-hard quartz fibers, oriented parallel to the beam direction. The calorimeter towers in the barrel region have segmentation of Δη×Δϕ=0.087×0.087, becoming progressively larger in the endcap and forward regions (Δη×Δϕ=0.175×0.175 at η∼4.5).
The CMS trigger system consists of a hardware Level-1 trigger and a software high-level trigger. Jets formed online by the trigger system use ECAL, HCAL and HF inputs for energy clustering and are not corrected for the jet energy response. The minimum-bias trigger was defined as the coincidence of a signal from one of the two beam scintillation counters covering the range 3.23<|η|<4.65 and a signal from one of the beam pick-up timing devices.
The data were collected in 2010, when LHC collided protons at \(\sqrt{s}=7\text{~TeV}\). Various triggers were used to cover the largest possible range in rapidity separation between jets. Dijets with moderate |Δy| were selected by a single-jet trigger with a threshold on the raw (uncorrected) jet transverse momentum of 15 GeV. This trigger was significantly prescaled as the instantaneous luminosity increased, and the effective integrated luminosity recorded with it is ≃33 nb−1.
Dijet events with large rapidity separation are rare. Therefore, a dedicated trigger for forward–backward dijets was developed. This forward–backward–dijet trigger selects events with two jets in opposite hemispheres and |η|>3.0, and jet raw transverse momentum p T>15 GeV. It was operated with moderate prescaling, and the effective integrated luminosity recorded with it is ≃5 pb−1. This allowed to collect a number of dijet events at high |Δy| values more than 100 times larger than with the single-jet trigger.
The trigger efficiency was measured by means of a control sample selected with the minimum-bias trigger. The single-jet trigger was found to be 100 % efficient for dijets with corrected p T>35 GeV. The single-jet trigger was also used for the determination of the efficiency of the forward–backward–dijet trigger. The latter was 100 % efficient for dijets with p T>35 GeV.
Jets were reconstructed offline from the energy depositions in the calorimeter towers, clustered with the anti-k T algorithm [27, 28] with a distance parameter R=0.5. In the reconstruction process, the contribution from each tower was assigned a momentum, the absolute value and the direction of which were given by the energy measured in the tower, and the coordinates of the tower, respectively. The raw jet energy was obtained from the sum of the tower energies, and the raw jet momentum from the vectorial sum of the tower momenta. The raw jet energies were then corrected to establish a uniform relative response of the calorimeter in η and a calibrated absolute response in transverse momentum p T [29]. The jet energy resolution for calorimeter jets with p T∼35 GeV is about 22 % for |η|<0.5 and about 10 % for 4<|η|<4.5 [30]. The uncertainty of the jet energy calibration for jets with p T∼35 GeV depends on η and is ≃7–8 % [29].
In the offline analysis, at least one well-reconstructed primary vertex is required to be present within ±24 cm of the nominal interaction point along the beamline [31]. In order to reduce the sensitivity to overlapping pp collisions (the so-called “pile-up” events), events with only one primary vertex reconstructed within the luminous region were used for the measurement.
Loose jet quality cuts [32] were used to suppress the effect of calorimeter noise. Events with at least two jets with p T>35 GeV and |y|<4.7 were selected; only jets satisfying these criteria were used for the analysis. All pairwise combinations of jets from the selected events entered the inclusive distribution. For studies of Mueller–Navelet jets, only the pair consisting of the most forward and the most backward jet was considered. The exclusive dijet sample is a subset of the inclusive and Mueller–Navelet samples, and consists of events where exactly one pair of jets is found. The measured observables, R incl and R MN, are defined as the ratios of the yield of inclusive or Mueller–Navelet dijets to the yield of exclusive dijets in a specific |Δy| bin, respectively.
Detector effects were accounted for by applying bin-by-bin corrections derived from Monte Carlo (MC) simulations. Simulated events produced with the generators pythia6 (version 6.422) tune Z2 [33–35] and herwig++ [36] (version 2.4.2 with default settings) were passed through the full CMS detector simulation based on the Geant4 package [37], and were input to the same event-reconstruction program as used for the data. To quantify detector effects, the distributions obtained from detector-level quantities were compared to the distributions obtained at the level of stable particles (lifetime τ such that cτ>10 mm). The ratios of the stable-particle level and detector-level quantities in a given bin were used to correct the data.
Because of the finite p T and y resolutions, the detector-level distributions deviate from the corresponding ones at stable-particle level. Events can migrate to and from the exclusive or the inclusive samples because of fluctuations of the measured transverse jet momentum around the \(p_{\mathrm{T}}^{\text{min}}\) threshold. The amount of these migrations was estimated not to exceed 20 %. Similarly, the |Δy| value measured at detector level may fall into a different |Δy| bin compared to the stable-particle level; this effect, for the present data, is typically around 5–10 % and reaches 15–25 % at most. The influence of these migrations on the measured ratios R incl and R MN is minimal as the effects for numerator and denominator are similar. The uncorrected R incl and R MN ratios are reasonably well reproduced by the pythia6 events and less well by herwig++ events, both of which have been passed through the detector simulation. The p T and η distributions of the jets at the detector level for both MC generators agree with the data within the jet energy scale uncertainty (see also [2]). The correction factors were therefore obtained with pythia6, while their model dependence was estimated from the difference between the pythia6 and herwig++ corrections.
The following sources of systematic effects were considered:
-
(1)
Uncertainty of the jet energy calibration. The uncertainty of the measurement was estimated by shifting the jet energy scale (JES) by the p T- and η-dependent uncertainties derived in [29]. The resulting variation of the measurements does not exceed 4.2 % for R incl and 3.8 % for R MN.
-
(2)
Uncertainty of the corrections for detector effects.
-
(a)
Uncertainty due to model dependence of the correction factors. As discussed above, correction factors were determined by using herwig++ and pythia6, and corrected measurements were obtained for each case. The difference in the results was taken as a measure of the model dependence of the correction factors; it does not exceed 3.4 % for R incl and 3.3 % for R MN.
-
(b)
Uncertainty related to the quality of the MC description of the jet p T and y resolutions. These resolutions were modified by ±10 % in the simulation as recommended in [30]. A conservative estimate of the uncertainty associated to this effect does not exceed ±1.0 %.
-
(a)
-
(3)
An additional systematic effect may originate from the extra energy and jets due to pile-up collisions. As noted earlier, the measurement was restricted to events with only one reconstructed primary vertex to reduce the impact of pile-up. However, a contribution from interactions undetected because of vertex reconstruction inefficiency may still be present. By comparing data taken at different instantaneous luminosities, an upper limit of 1.3 % was estimated for this effect. The value was obtained for pairs of jets in the forward region, where the impact of pile-up on jet reconstruction is more significant [29]; the effect is thus expected to be smaller for jets at central rapidities.
The total systematic uncertainty was calculated as the quadratic sum of the individual uncertainties. The total uncertainty is |Δy| dependent but always smaller than 5.6 % for R incl and 4.8 % for R MN (Table 1).
The measured ratios, corrected for detector effects, were compared to the predictions of several MC generators at the stable-particle level. A DGLAP leading-order parton shower approach is used in pythia6 (version 6.422) tune Z2 [35], pythia8 (version 8.145) [38] tune 4C [39] and herwig++ (version 2.5.1) tune UE-7000-EE-3 [36, 40]. For the simulation of the non-perturbative fragmentation, pythia uses the Lund string model, and herwig++ the cluster fragmentation approach. The tunes mentioned above include multiple parton interactions (MPI) as a part of the underlying event (UE) modeling. The dijet observables might be affected by MPI through jets that do not originate from the same hard interaction. The effect was estimated by switching off the MPI options in pythia6 and herwig++; no significant change in the predictions was observed.
The Monte Carlo generators cascade (version 2.2.03) [41] and hej (version 1.3.2) [42] are motivated by the leading-logarithmic BFKL approach and incorporate parts of a next-to-leading logarithmic approximation. The hej generator produces parton-level jets; the corresponding showers were produced with the ariadne program [43]. The hej+ariadne package [44] version 0.99b, consisting of hej 1.3.2 and ariadne 4.12, was used.
The ratio R incl of inclusive to exclusive dijet production as a function of |Δy| is presented in Fig. 1 (top panel). On average the inclusive cross section is 1.2–1.5 times larger than the exclusive cross section. The ratio R incl grows with increasing |Δy|, as expected because of the larger phase space for hard parton radiation. At the highest |Δy|, R incl is expected to decrease because energy-momentum conservation suppresses the emission of extra jets. The |Δy| value where R incl starts to decrease varies from one MC generator to another, as can be seen in Fig. 1.
Ratios of the inclusive to exclusive dijet cross sections as a function of the rapidity separation |Δy| between the two jets, R incl (top panel) and R MN (bottom panel), compared to the predictions of the DGLAP-based MC generators pythia6, pythia8 and herwig++, as well as of cascade and hej+ariadne, which incorporate elements of the BFKL approach. The shaded band indicates the size of the total systematic uncertainty of the data. Statistical uncertainties are smaller than the symbol sizes. Because of limitations in the cascade generator, it was not possible to obtain a reliable prediction for |Δy|>8
The predictions from pythia6 and pythia8 agree with the measurement. herwig++ overestimates the ratio R incl at medium and large rapidity intervals. A detailed comparison between the data and the predictions of the DGLAP-based MC generators is presented as a ratio in Fig. 2 (top panel). It was checked explicitly that the results obtained from pythia6 and herwig++ at parton level are close to the corresponding ones at stable-particle level. The different behaviour of pythia6 and herwig++ is also observed at parton level.
Predictions for R incl (top) and R MN (bottom) from DGLAP-based MC generators presented as ratio to data corrected for detector effects. Both BFKL-motivated generators cascade and hej+ariadne (not shown) lead to a MC/data ratio well above unity. The shaded band indicates the size of the total systematic uncertainty of the data while statistical uncertainties are shown as bars
The ratio R MN and the corresponding MC to data ratio are presented in the bottom panels of Figs. 1–2. At large |Δy|, R MN approaches R incl as extra jet radiation contributing to R incl tends to concentrate at moderate rapidities. The quality of the predictions of the DGLAP-based MC generators for R MN is similar to those for R incl. The MC generators cascade and hej+ariadne considerably overestimate the measurements of both R incl and R MN.
The ATLAS Collaboration measured several observables for dijets as functions of the rapidity separation between jets [21]. One is the fraction of events without jets with p T above \(p_{\mathrm{T}}^{\mathrm{veto}}\) in the rapidity interval between the most forward and the most backward jets, which is equal to 1/R MN. A difference between data and the parton-level HEJ prediction at large |Δy| for 70<〈p T〉<90 GeV, \(p_{\mathrm{T}}^{\mathrm{veto}}=\langle p_{\mathrm{T}}\rangle\) and |Δy|<6 was reported (Fig. 8 from [21]), which is in qualitative agreement with the result presented here.
To conclude, the first measurement of the ratios R incl and R MN in a wide range of rapidity separation, up to |Δy|=9.2, in proton–proton collisions at \(\sqrt {s}=7\text{~TeV}\) was presented. A moderate rise of the ratio of the inclusive to exclusive dijet production cross sections as a function of |Δy| is observed. The predictions of the pythia6 and pythia8 generators agree with the measurements. The predictions of the herwig++ generator are larger than the measurement especially at large |Δy|. The BFKL-motivated generators cascade and hej+ariadne predict for these ratios a significantly stronger rise than observed. The moderate rise of the measured dijet ratios indicates that the BFKL effects are not dominant for jets with p T>35 GeV at the present collision energy of 7 TeV.
References
CMS Collaboration, Measurement of the inclusive jet cross section in pp collisions at \(\sqrt{s} = 7\mbox{~TeV}\). Phys. Rev. Lett. 107, 132001 (2011). doi:10.1103/PhysRevLett.107.132001, arXiv:1106.0208
CMS Collaboration, Measurement of the inclusive production cross sections for forward jets and for dijet events with one forward and one central jet in pp collisions at \(\sqrt{s} = 7\mbox{~TeV}\). J. High Energy Phys. 1206, 036 (2012). doi:10.1007/JHEP06(2012)036, arXiv:1202.0704
V.N. Gribov, L.N. Lipatov, Deep inelastic ep scattering in perturbation theory. Sov. J. Nucl. Phys. 15, 438 (1972)
V.N. Gribov, L.N. Lipatov, e + e − pair annihilation and deep inelastic ep scattering in perturbation theory. Sov. J. Nucl. Phys. 15, 675 (1972)
L.N. Lipatov, The parton model and perturbation theory. Sov. J. Nucl. Phys. 20, 94 (1975)
G. Altarelli, G. Parisi, Asymptotic freedom in parton language. Nucl. Phys. B 126, 298 (1977). doi:10.1016/0550-3213(77)90384-4
Y.L. Dokshitzer, Calculation of the structure functions for deep inelastic scattering and e + e − annihilation by perturbation theory in quantum chromodynamics. Sov. Phys. JETP 46, 641 (1977)
CMS Collaboration, Measurement of the differential dijet production cross section in proton–proton collisions at \(\sqrt{s} = 7\mbox{~TeV}\). Phys. Lett. B 700, 187 (2011). doi:10.1016/j.physletb.2011.05.027, arXiv:1104.1693
E.A. Kuraev, L.N. Lipatov, V.S. Fadin, Multi-reggeon processes in the Yang–Mills theory. Sov. Phys. JETP 44, 443 (1976)
E.A. Kuraev, L.N. Lipatov, V.S. Fadin, The Pomeranchuk singularity in nonabelian gauge theories. Sov. Phys. JETP 45, 199 (1977)
I.I. Balitsky, L.N. Lipatov, The Pomeranchuk singularity in quantum chromodynamics. Sov. J. Nucl. Phys. 28, 822 (1978)
E.M. Levin et al., Heavy quark production in semihard nucleon interactions. Sov. J. Nucl. Phys. 53, 657 (1991)
S. Catani, M. Ciafaloni, F. Hautmann, High-energy factorization and small-x heavy flavour production. Nucl. Phys. B 366, 135 (1991). doi:10.1016/0550-3213(91)90055-3
J.C. Collins, R.K. Ellis, Heavy quark production in very high-energy hadron collisions. Nucl. Phys. B 360, 3 (1991). doi:10.1016/0550-3213(91)90288-9
L. Lipatov, Small x physics in perturbative QCD. Phys. Rep. 286, 131–198 (1997). doi:10.1016/S0370-1573(96)00045-2, arXiv:hep-ph/9610276
V.T. Kim, G.B. Pivovarov, BFKL QCD pomeron in high-energy hadron collisions: inclusive dijet production. Phys. Rev. D 53, 6 (1996). doi:10.1103/PhysRevD.53.R6, arXiv:hep-ph/9506381
A.H. Mueller, H. Navelet, An inclusive minijet cross-section and the bare pomeron in QCD. Nucl. Phys. B 282, 727 (1987). doi:10.1016/0550-3213(87)90705-X
B. Andersson et al., Small x phenomenology: summary and status. Eur. Phys. J. C 25, 77 (2002). doi:10.1007/s10052-002-0998-7, arXiv:hep-ph/0204115
D0 Collaboration, The azimuthal decorrelation of jets widely separated in rapidity. Phys. Rev. Lett. 77, 595 (1996). doi:10.1103/PhysRevLett.77.595, arXiv:hep-ex/9603010
D0 Collaboration, Probing BFKL dynamics in the dijet cross section at large rapidity intervals in \(p\bar{p}\) collisions at \(\sqrt{s} = 1800\mbox{~GeV and 630-GeV}\). Phys. Rev. Lett. 84, 5722 (2000). doi:10.1103/PhysRevLett.84.5722, arXiv:hep-ex/9912032
ATLAS Collaboration, Measurement of dijet production with a veto on additional central jet activity in pp collisions with \(\sqrt{s}=7\mbox{~TeV}\) using the ATLAS detector. J. High Energy Phys. 09, 053 (2011). doi:10.1007/JHEP09(2011)053, arXiv:1107.1641v2.
ZEUS Collaboration, Forward jet production in deep inelastic scattering at HERA. Eur. Phys. J. C 6, 239 (1999). doi:10.1007/s100529801018, arXiv:hep-ex/9805016v1
ZEUS Collaboration, Forward-jet production in deep inelastic ep scattering at HERA. Eur. Phys. J. C 52, 515 (2007). doi:10.1140/epjc/s10052-007-0418-0, arXiv:0707.3093
H1 Collaboration, Transverse energy and forward jet production in the low x regime at HERA. Phys. Lett. B 356, 118 (1995). doi:10.1016/0370-2693(95)00804-T
H1 Collaboration, Measurement of the azimuthal correlation between the most forward jet and the scattered positron in deep-inelastic scattering at HERA. Eur. Phys. J. C 52, 1910 (2012). doi:10.1140/epjc/s10052-012-1910-8, arXiv:1111.4227
CMS Collaboration, The CMS experiment at the CERN LHC. J. Instrum. 03, S08004 (2008). doi:10.1088/1748-0221/3/08/S08004
M. Cacciari, G.P. Salam, G. Soyez, The anti-kt jet clustering algorithm. J. High Energy Phys. 04, 063 (2008). doi:10.1088/1126-6708/2008/04/063, arXiv:0802.1189
M. Cacciari, G.P. Salam, Dispelling the N 3 myth for the kT jet-finder. Phys. Lett. B 641, 57 (2006). doi:10.1016/j.physletb.2006.08.037, arXiv:hep-ph/0512210
CMS Collaboration, Determination of jet energy calibration and transverse momentum resolution in CMS. J. Instrum. 6, P11002 (2011). doi:10.1088/1748-0221/6/11/P11002, arXiv:1107.4277
CMS Collaboration, Jet performance in pp collisions at \(\sqrt{s}=7\mbox{~TeV}\), CMS physics analysis summary CMS-PAS-JME-10-003 (2010)
CMS Collaboration, Tracking and primary vertex results in first 7 TeV collisions, CMS physics analysis summary CMS-PAS-TRK-10-005 (2010)
CMS Collaboration, Calorimeter jet quality criteria for the first CMS collision data, CMS physics analysis summary CMS-PAS-JME-09-008 (2010)
T. Sjöstrand, S. Mrenna, P. Skands, Pythia 6.4 physics and manual. J. High Energy Phys. 05, 026 (2006). doi:10.1088/1126-6708/2006/05/026
R. Field, Early LHC underlying event data—findings and surprises (2010). arXiv:1010.3558
CMS Collaboration, Measurement of the underlying event activity at the LHC with \(\sqrt{s} = 7\mbox{~TeV}\) and comparison with \(\sqrt{s} = 0.9\mbox{~TeV}\). J. High Energy Phys. 09, 109 (2011). doi:10.1007/JHEP09(2011)109, arXiv:1107.0330
M. Bähr et al., HERWIG++ physics and manual. Eur. Phys. J. C 58, 639 (2008). doi:10.1140/epjc/s10052-008-0798-9
S. Agostinelli et al., Geant4—a simulation toolkit. Nucl. Instrum. Methods A 506, 250 (2003). doi:10.1016/S0168-9002(03)01368-8
T. Sjöstrand, S. Mrenna, P. Skands, A brief introduction to PYTHIA 8.1. Comput. Phys. Commun. 178, 852 (2008). doi:10.1016/j.cpc.2008.01.036
R. Corke, T. Sjöstrand, Interleaved parton showers and tuning prospects. J. High Energy Phys. 3, 32 (2011). doi:10.1007/JHEP03(2011)032, arXiv:1011.1759
S. Gieseke et al., HERWIG++ 2.5 release note, arXiv:1102.1672v1
H. Jung et al., The CCFM Monte Carlo generator CASCADE version 2.2.03. Eur. Phys. J. C 70, 1237 (2010). doi:10.1140/epjc/s10052-010-1507-z, arXiv:1008.0152
J.R. Andersen, J.M. Smillie, Multiple jets at the LHC with high energy jets. J. High Energy Phys. 06, 010 (2011). doi:10.1007/JHEP06(2011)010, arXiv:1101.5394
L. Lönnblad, Ariadne version 4: a program for simulation of QCD cascades implementing the colour dipole model. Comput. Phys. Commun. 71, 15 (1992). doi:10.1016/0010-4655(92)90068-A
J. Andersen, L. Lonnblad, J. Smillie, A parton shower for high energy jets. J. High Energy Phys. 7, 110 (2011). doi:10.1007/JHEP07(2011)110, arXiv:1104.1316
Acknowledgements
We congratulate our colleagues in the CERN accelerator departments for the excellent performance of the LHC machine. We thank the technical and administrative staff at CERN and other CMS institutes, and acknowledge support from: FMSR (Austria); FNRS and FWO (Belgium); CNPq, CAPES, FAPERJ, and FAPESP (Brazil); MES (Bulgaria); CERN; CAS, MoST, and NSFC (China); COLCIENCIAS (Colombia); MSES (Croatia); RPF (Cyprus); MoER, SF0690030s09 and ERDF (Estonia); Academy of Finland, MEC, and HIP (Finland); CEA and CNRS/IN2P3 (France); BMBF, DFG, and HGF (Germany); GSRT (Greece); OTKA and NKTH (Hungary); DAE and DST (India); IPM (Iran); SFI (Ireland); INFN (Italy); NRF and WCU (Korea); LAS (Lithuania); CINVESTAV, CONACYT, SEP, and UASLP-FAI (Mexico); MSI (New Zealand); PAEC (Pakistan); MSHE and NSC (Poland); FCT (Portugal); JINR (Armenia, Belarus, Georgia, Ukraine, Uzbekistan); MON, RosAtom, RAS and RFBR (Russia); MSTD (Serbia); MICINN and CPAN (Spain); Swiss Funding Agencies (Switzerland); NSC (Taipei); TUBITAK and TAEK (Turkey); STFC (United Kingdom); DOE and NSF (USA).
Open Access
This article is distributed under the terms of the Creative Commons Attribution License which permits any use, distribution, and reproduction in any medium, provided the original author(s) and the source are credited.
Author information
Authors and Affiliations
Yerevan Physics Institute, Yerevan, Armenia
S. Chatrchyan, V. Khachatryan, A. M. Sirunyan & A. Tumasyan
Institut für Hochenergiephysik der OeAW, Wien, Austria
W. Adam, T. Bergauer, M. Dragicevic, J. Erö, C. Fabjan, M. Friedl, R. Frühwirth, V. M. Ghete, J. Hammer, M. Hoch, N. Hörmann, J. Hrubec, M. Jeitler, W. Kiesenhofer, M. Krammer, D. Liko, I. Mikulec, M. Pernicka, B. Rahbaran, C. Rohringer, H. Rohringer, R. Schöfbeck, J. Strauss, A. Taurok, F. Teischinger, P. Wagner, W. Waltenberger, G. Walzel, E. Widl & C.-E. Wulz
National Centre for Particle and High Energy Physics, Minsk, Belarus
V. Mossolov, N. Shumeiko & J. Suarez Gonzalez
Universiteit Antwerpen, Antwerpen, Belgium
S. Bansal, L. Benucci, T. Cornelis, E. A. De Wolf, X. Janssen, S. Luyckx, T. Maes, L. Mucibello, S. Ochesanu, B. Roland, R. Rougny, M. Selvaggi, H. Van Haevermaet, P. Van Mechelen, N. Van Remortel & A. Van Spilbeeck
Vrije Universiteit Brussel, Brussel, Belgium
F. Blekman, S. Blyweert, J. D’Hondt, R. Gonzalez Suarez, A. Kalogeropoulos, M. Maes, A. Olbrechts, W. Van Doninck, P. Van Mulders, G. P. Van Onsem & I. Villella
Université Libre de Bruxelles, Bruxelles, Belgium
O. Charaf, B. Clerbaux, G. De Lentdecker, V. Dero, A. P. R. Gay, G. H. Hammad, T. Hreus, A. Léonard, P. E. Marage, L. Thomas, C. Vander Velde, P. Vanlaer & J. Wickens
Ghent University, Ghent, Belgium
V. Adler, K. Beernaert, A. Cimmino, S. Costantini, G. Garcia, M. Grunewald, B. Klein, J. Lellouch, A. Marinov, J. Mccartin, A. A. Ocampo Rios, D. Ryckbosch, N. Strobbe, F. Thyssen, M. Tytgat, L. Vanelderen, P. Verwilligen, S. Walsh, E. Yazgan & N. Zaganidis
Université Catholique de Louvain, Louvain-la-Neuve, Belgium
S. Basegmez, G. Bruno, L. Ceard, J. De Favereau De Jeneret, C. Delaere, T. du Pree, D. Favart, L. Forthomme, A. Giammanco, G. Grégoire, J. Hollar, V. Lemaitre, J. Liao, O. Militaru, C. Nuttens, D. Pagano, A. Pin, K. Piotrzkowski & N. Schul
Université de Mons, Mons, Belgium
N. Beliy, T. Caebergs & E. Daubie
Centro Brasileiro de Pesquisas Fisicas, Rio de Janeiro, Brazil
G. A. Alves, M. Correa Martins Junior, D. De Jesus Damiao, T. Martins, M. E. Pol & M. H. G. Souza
Universidade do Estado do Rio de Janeiro, Rio de Janeiro, Brazil
W. L. Aldá Júnior, W. Carvalho, A. Custódio, E. M. Da Costa, C. De Oliveira Martins, S. Fonseca De Souza, D. Matos Figueiredo, L. Mundim, H. Nogima, V. Oguri, W. L. Prado Da Silva, A. Santoro, S. M. Silva Do Amaral, L. Soares Jorge & A. Sznajder
Instituto de Fisica Teorica, Universidade Estadual Paulista, Sao Paulo, Brazil
T. S. Anjos, C. A. Bernardes, F. A. Dias, T. R. Fernandez Perez Tomei, E. M. Gregores, C. Lagana, F. Marinho, P. G. Mercadante, S. F. Novaes & Sandra S. Padula
Institute for Nuclear Research and Nuclear Energy, Sofia, Bulgaria
V. Genchev, P. Iaydjiev, S. Piperov, M. Rodozov, S. Stoykova, G. Sultanov, V. Tcholakov, R. Trayanov & M. Vutova
University of Sofia, Sofia, Bulgaria
A. Dimitrov, R. Hadjiiska, A. Karadzhinova, V. Kozhuharov, L. Litov, B. Pavlov & P. Petkov
Institute of High Energy Physics, Beijing, China
J. G. Bian, G. M. Chen, H. S. Chen, C. H. Jiang, D. Liang, S. Liang, X. Meng, J. Tao, J. Wang, J. Wang, X. Wang, Z. Wang, H. Xiao, M. Xu, J. Zang & Z. Zhang
State Key Lab. of Nucl. Phys. and Tech., Peking University, Beijing, China
C. Asawatangtrakuldee, Y. Ban, S. Guo, Y. Guo, W. Li, S. Liu, Y. Mao, S. J. Qian, H. Teng, S. Wang, B. Zhu & W. Zou
Universidad de Los Andes, Bogota, Colombia
A. Cabrera, B. Gomez Moreno, A. F. Osorio Oliveros & J. C. Sanabria
Technical University of Split, Split, Croatia
N. Godinovic, D. Lelas, R. Plestina, D. Polic & I. Puljak
University of Split, Split, Croatia
Z. Antunovic, M. Dzelalija & M. Kovac
Institute Rudjer Boskovic, Zagreb, Croatia
V. Brigljevic, S. Duric, K. Kadija, J. Luetic & S. Morovic
University of Cyprus, Nicosia, Cyprus
A. Attikis, M. Galanti, J. Mousa, C. Nicolaou, F. Ptochos & P. A. Razis
Charles University, Prague, Czech Republic
M. Finger & M. Finger Jr.
Academy of Scientific Research and Technology of the Arab Republic of Egypt, Egyptian Network of High Energy Physics, Cairo, Egypt
Y. Assran, A. Ellithi Kamel, S. Khalil, M. A. Mahmoud & A. Radi
National Institute of Chemical Physics and Biophysics, Tallinn, Estonia
A. Hektor, M. Kadastik, M. Müntel, M. Raidal, L. Rebane & A. Tiko
Department of Physics, University of Helsinki, Helsinki, Finland
V. Azzolini, P. Eerola, G. Fedi & M. Voutilainen
Helsinki Institute of Physics, Helsinki, Finland
S. Czellar, J. Härkönen, A. Heikkinen, V. Karimäki, R. Kinnunen, M. J. Kortelainen, T. Lampén, K. Lassila-Perini, S. Lehti, T. Lindén, P. Luukka, T. Mäenpää, T. Peltola, E. Tuominen, J. Tuominiemi, E. Tuovinen, D. Ungaro & L. Wendland
Lappeenranta University of Technology, Lappeenranta, Finland
K. Banzuzi, A. Korpela & T. Tuuva
Laboratoire d’Annecy-le-Vieux de Physique des Particules, IN2P3-CNRS, Annecy-le-Vieux, France
D. Sillou
DSM/IRFU, CEA/Saclay, Gif-sur-Yvette, France
M. Besancon, S. Choudhury, M. Dejardin, D. Denegri, B. Fabbro, J. L. Faure, F. Ferri, S. Ganjour, A. Givernaud, P. Gras, G. Hamel de Monchenault, P. Jarry, E. Locci, J. Malcles, L. Millischer, J. Rander, A. Rosowsky, I. Shreyber & M. Titov
Laboratoire Leprince-Ringuet, Ecole Polytechnique, IN2P3-CNRS, Palaiseau, France
S. Baffioni, F. Beaudette, L. Benhabib, L. Bianchini, M. Bluj, C. Broutin, P. Busson, C. Charlot, N. Daci, T. Dahms, L. Dobrzynski, S. Elgammal, R. Granier de Cassagnac, M. Haguenauer, P. Miné, C. Mironov, C. Ochando, P. Paganini, D. Sabes, R. Salerno, Y. Sirois, C. Thiebaux, C. Veelken & A. Zabi
Institut Pluridisciplinaire Hubert Curien, Université de Strasbourg, Université de Haute Alsace Mulhouse, CNRS/IN2P3, Strasbourg, France
J.-L. Agram, J. Andrea, D. Bloch, D. Bodin, J.-M. Brom, M. Cardaci, E. C. Chabert, C. Collard, E. Conte, F. Drouhin, C. Ferro, J.-C. Fontaine, D. Gelé, U. Goerlach, P. Juillot, M. Karim, A.-C. Le Bihan & P. Van Hove
Centre de Calcul de l’Institut National de Physique Nucleaire et de Physique des Particules (IN2P3), Villeurbanne, France
F. Fassi & D. Mercier
Université de Lyon, Université Claude Bernard Lyon 1, CNRS-IN2P3, Institut de Physique Nucléaire de Lyon, Villeurbanne, France
C. Baty, S. Beauceron, N. Beaupere, M. Bedjidian, O. Bondu, G. Boudoul, D. Boumediene, H. Brun, J. Chasserat, R. Chierici, D. Contardo, P. Depasse, H. El Mamouni, A. Falkiewicz, J. Fay, S. Gascon, M. Gouzevitch, B. Ille, T. Kurca, T. Le Grand, M. Lethuillier, L. Mirabito, S. Perries, V. Sordini, S. Tosi, Y. Tschudi, P. Verdier & S. Viret
Institute of High Energy Physics and Informatization, Tbilisi State University, Tbilisi, Georgia
D. Lomidze
I. Physikalisches Institut, RWTH Aachen University, Aachen, Germany
G. Anagnostou, S. Beranek, M. Edelhoff, L. Feld, N. Heracleous, O. Hindrichs, R. Jussen, K. Klein, J. Merz, A. Ostapchuk, A. Perieanu, F. Raupach, J. Sammet, S. Schael, D. Sprenger, H. Weber, B. Wittmer & V. Zhukov
III. Physikalisches Institut A, RWTH Aachen University, Aachen, Germany
M. Ata, J. Caudron, E. Dietz-Laursonn, M. Erdmann, A. Güth, T. Hebbeker, C. Heidemann, K. Hoepfner, T. Klimkovich, D. Klingebiel, P. Kreuzer, D. Lanske, J. Lingemann, C. Magass, M. Merschmeyer, A. Meyer, M. Olschewski, P. Papacz, H. Pieta, H. Reithler, S. A. Schmitz, L. Sonnenschein, J. Steggemann, D. Teyssier & M. Weber
III. Physikalisches Institut B, RWTH Aachen University, Aachen, Germany
M. Bontenackels, V. Cherepanov, M. Davids, G. Flügge, H. Geenen, M. Geisler, W. Haj Ahmad, F. Hoehle, B. Kargoll, T. Kress, Y. Kuessel, A. Linn, A. Nowack, L. Perchalla, O. Pooth, J. Rennefeld, P. Sauerland, A. Stahl & M. H. Zoeller
Deutsches Elektronen-Synchrotron, Hamburg, Germany
M. Aldaya Martin, W. Behrenhoff, U. Behrens, M. Bergholz, A. Bethani, K. Borras, A. Burgmeier, A. Cakir, L. Calligaris, A. Campbell, E. Castro, D. Dammann, G. Eckerlin, D. Eckstein, A. Flossdorf, G. Flucke, A. Geiser, J. Hauk, H. Jung, M. Kasemann, P. Katsas, C. Kleinwort, H. Kluge, A. Knutsson, M. Krämer, D. Krücker, E. Kuznetsova, W. Lange, W. Lohmann, B. Lutz, R. Mankel, I. Marfin, M. Marienfeld, I.-A. Melzer-Pellmann, A. B. Meyer, J. Mnich, A. Mussgiller, S. Naumann-Emme, J. Olzem, A. Petrukhin, D. Pitzl, A. Raspereza, P. M. Ribeiro Cipriano, M. Rosin, J. Salfeld-Nebgen, R. Schmidt, T. Schoerner-Sadenius, N. Sen, A. Spiridonov, M. Stein, J. Tomaszewska, R. Walsh & C. Wissing
University of Hamburg, Hamburg, Germany
C. Autermann, V. Blobel, S. Bobrovskyi, J. Draeger, H. Enderle, J. Erfle, U. Gebbert, M. Görner, T. Hermanns, R. S. Höing, K. Kaschube, G. Kaussen, H. Kirschenmann, R. Klanner, J. Lange, B. Mura, F. Nowak, N. Pietsch, C. Sander, H. Schettler, P. Schleper, E. Schlieckau, A. Schmidt, M. Schröder, T. Schum, H. Stadie, G. Steinbrück & J. Thomsen
Institut für Experimentelle Kernphysik, Karlsruhe, Germany
C. Barth, J. Berger, T. Chwalek, W. De Boer, A. Dierlamm, G. Dirkes, M. Feindt, J. Gruschke, M. Guthoff, C. Hackstein, F. Hartmann, M. Heinrich, H. Held, K. H. Hoffmann, S. Honc, I. Katkov, J. R. Komaragiri, T. Kuhr, D. Martschei, S. Mueller, Th. Müller, M. Niegel, A. Nürnberg, O. Oberst, A. Oehler, J. Ott, T. Peiffer, G. Quast, K. Rabbertz, F. Ratnikov, N. Ratnikova, M. Renz, S. Röcker, C. Saout, A. Scheurer, P. Schieferdecker, F.-P. Schilling, M. Schmanau, G. Schott, H. J. Simonis, F. M. Stober, D. Troendle, J. Wagner-Kuhr, T. Weiler, M. Zeise & E. B. Ziebarth
Institute of Nuclear Physics “Demokritos”, Aghia Paraskevi, Greece
G. Daskalakis, T. Geralis, S. Kesisoglou, A. Kyriakis, D. Loukas, I. Manolakos, A. Markou, C. Markou, C. Mavrommatis & E. Ntomari
University of Athens, Athens, Greece
L. Gouskos, T. J. Mertzimekis, A. Panagiotou, N. Saoulidou & E. Stiliaris
University of Ioánnina, Ioánnina, Greece
I. Evangelou, C. Foudas, P. Kokkas, N. Manthos, I. Papadopoulos, V. Patras & F. A. Triantis
KFKI Research Institute for Particle and Nuclear Physics, Budapest, Hungary
A. Aranyi, G. Bencze, L. Boldizsar, C. Hajdu, P. Hidas, D. Horvath, A. Kapusi, K. Krajczar, F. Sikler, V. Veszpremi & G. Vesztergombi
Institute of Nuclear Research ATOMKI, Debrecen, Hungary
N. Beni, J. Molnar, J. Palinkas & Z. Szillasi
University of Debrecen, Debrecen, Hungary
J. Karancsi, P. Raics, Z. L. Trocsanyi & B. Ujvari
Panjab University, Chandigarh, India
S. B. Beri, V. Bhatnagar, N. Dhingra, R. Gupta, M. Jindal, M. Kaur, J. M. Kohli, M. Z. Mehta, N. Nishu, L. K. Saini, A. Sharma, A. P. Singh, J. Singh & S. P. Singh
University of Delhi, Delhi, India
S. Ahuja, B. C. Choudhary, A. Kumar, A. Kumar, S. Malhotra, M. Naimuddin, K. Ranjan, V. Sharma & R. K. Shivpuri
Saha Institute of Nuclear Physics, Kolkata, India
S. Banerjee, S. Bhattacharya, S. Dutta, B. Gomber, Sa. Jain, Sh. Jain, R. Khurana & S. Sarkar
Bhabha Atomic Research Centre, Mumbai, India
R. K. Choudhury, D. Dutta, S. Kailas, V. Kumar, A. K. Mohanty, L. M. Pant & P. Shukla
Tata Institute of Fundamental Research - EHEP, Mumbai, India
T. Aziz, S. Ganguly, M. Guchait, A. Gurtu, M. Maity, G. Majumder, K. Mazumdar, G. B. Mohanty, B. Parida, A. Saha, K. Sudhakar & N. Wickramage
Tata Institute of Fundamental Research - HECR, Mumbai, India
S. Banerjee, S. Dugad & N. K. Mondal
Institute for Research in Fundamental Sciences (IPM), Tehran, Iran
H. Arfaei, H. Bakhshiansohi, S. M. Etesami, A. Fahim, M. Hashemi, H. Hesari, A. Jafari, M. Khakzad, A. Mohammadi, M. Mohammadi Najafabadi, S. Paktinat Mehdiabadi, B. Safarzadeh & M. Zeinali
INFN Sezione di Bari, Bari, Italy
M. Abbrescia, L. Barbone, C. Calabria, S. S. Chhibra, A. Colaleo, D. Creanza, N. De Filippis, M. De Palma, L. Fiore, G. Iaselli, L. Lusito, G. Maggi, M. Maggi, N. Manna, B. Marangelli, S. My, S. Nuzzo, N. Pacifico, A. Pompili, G. Pugliese, F. Romano, G. Selvaggi, L. Silvestris, G. Singh, S. Tupputi & G. Zito
Università di Bari, Bari, Italy
M. Abbrescia, L. Barbone, C. Calabria, S. S. Chhibra, M. De Palma, L. Lusito, N. Manna, B. Marangelli, S. Nuzzo, N. Pacifico, A. Pompili, G. Selvaggi, G. Singh & S. Tupputi
Politecnico di Bari, Bari, Italy
D. Creanza, N. De Filippis, G. Iaselli, G. Maggi, S. My, G. Pugliese & F. Romano
INFN Sezione di Bologna, Bologna, Italy
G. Abbiendi, A. C. Benvenuti, D. Bonacorsi, S. Braibant-Giacomelli, L. Brigliadori, P. Capiluppi, A. Castro, F. R. Cavallo, M. Cuffiani, G. M. Dallavalle, F. Fabbri, A. Fanfani, D. Fasanella, P. Giacomelli, C. Grandi, S. Marcellini, G. Masetti, M. Meneghelli, A. Montanari, F. L. Navarria, F. Odorici, A. Perrotta, F. Primavera, A. M. Rossi, T. Rovelli, G. Siroli & R. Travaglini
Università di Bologna, Bologna, Italy
S. Braibant-Giacomelli, P. Capiluppi, A. Castro, M. Cuffiani, A. Fanfani, M. Meneghelli, F. L. Navarria, A. M. Rossi, T. Rovelli, G. Siroli & R. Travaglini
INFN Sezione di Catania, Catania, Italy
S. Albergo, G. Cappello, M. Chiorboli, S. Costa, R. Potenza, A. Tricomi & C. Tuve
Università di Catania, Catania, Italy
S. Albergo, G. Cappello, M. Chiorboli, S. Costa, R. Potenza, A. Tricomi & C. Tuve
INFN Sezione di Firenze, Firenze, Italy
G. Barbagli, V. Ciulli, C. Civinini, R. D’Alessandro, E. Focardi, S. Frosali, E. Gallo, S. Gonzi, M. Meschini, S. Paoletti, G. Sguazzoni & A. Tropiano
Università di Firenze, Firenze, Italy
V. Ciulli, R. D’Alessandro, E. Focardi, S. Frosali & S. Gonzi
INFN Laboratori Nazionali di Frascati, Frascati, Italy
L. Benussi, S. Bianco, S. Colafranceschi, F. Fabbri & D. Piccolo
INFN Sezione di Genova, Genova, Italy
P. Fabbricatore & R. Musenich
INFN Sezione di Milano-Bicocca, Milano, Italy
A. Benaglia, F. De Guio, L. Di Matteo, S. Fiorendi, S. Gennai, A. Ghezzi, S. Malvezzi, R. A. Manzoni, A. Martelli, A. Massironi, D. Menasce, L. Moroni, M. Paganoni, D. Pedrini, S. Ragazzi, N. Redaelli, S. Sala & T. Tabarelli de Fatis
Università di Milano-Bicocca, Milano, Italy
A. Benaglia, F. De Guio, L. Di Matteo, S. Fiorendi, A. Ghezzi, R. A. Manzoni, A. Martelli, A. Massironi, M. Paganoni, S. Ragazzi & T. Tabarelli de Fatis
INFN Sezione di Napoli, Napoli, Italy
S. Buontempo, C. A. Carrillo Montoya, N. Cavallo, A. De Cosa, O. Dogangun, F. Fabozzi, A. O. M. Iorio, L. Lista, M. Merola & P. Paolucci
Università di Napoli “Federico II”, Napoli, Italy
A. De Cosa, O. Dogangun & M. Merola
INFN Sezione di Padova, Padova, Italy
P. Azzi, N. Bacchetta, P. Bellan, D. Bisello, A. Branca, R. Carlin, P. Checchia, T. Dorigo, U. Dosselli, F. Gasparini, U. Gasparini, A. Gozzelino, K. Kanishchev, S. Lacaprara, I. Lazzizzera, M. Margoni, M. Mazzucato, A. T. Meneguzzo, M. Nespolo, L. Perrozzi, N. Pozzobon, P. Ronchese, F. Simonetto, E. Torassa, M. Tosi, A. Triossi, S. Vanini, P. Zotto & G. Zumerle
Università di Padova, Padova, Italy
P. Bellan, D. Bisello, R. Carlin, F. Gasparini, U. Gasparini, M. Margoni, A. T. Meneguzzo, N. Pozzobon, P. Ronchese, F. Simonetto, M. Tosi, S. Vanini, P. Zotto & G. Zumerle
Università di Trento (Trento), Padova, Italy
K. Kanishchev & I. Lazzizzera
INFN Sezione di Pavia, Pavia, Italy
U. Berzano, M. Gabusi, S. P. Ratti, C. Riccardi, P. Torre & P. Vitulo
Università di Pavia, Pavia, Italy
M. Gabusi, S. P. Ratti, C. Riccardi, P. Torre & P. Vitulo
INFN Sezione di Perugia, Perugia, Italy
M. Biasini, G. M. Bilei, B. Caponeri, L. Fanò, P. Lariccia, A. Lucaroni, G. Mantovani, M. Menichelli, A. Nappi, F. Romeo, A. Santocchia, S. Taroni & M. Valdata
Università di Perugia, Perugia, Italy
M. Biasini, B. Caponeri, L. Fanò, P. Lariccia, A. Lucaroni, G. Mantovani, A. Nappi, F. Romeo, A. Santocchia, S. Taroni & M. Valdata
INFN Sezione di Pisa, Pisa, Italy
P. Azzurri, G. Bagliesi, T. Boccali, G. Broccolo, R. Castaldi, R. T. D’Agnolo, R. Dell’Orso, F. Fiori, L. Foà, A. Giassi, A. Kraan, F. Ligabue, T. Lomtadze, L. Martini, A. Messineo, F. Palla, F. Palmonari, A. Rizzi, A. T. Serban, P. Spagnolo, R. Tenchini, G. Tonelli, A. Venturi & P. G. Verdini
Università di Pisa, Pisa, Italy
F. Fiori, A. Messineo, A. Rizzi & G. Tonelli
Scuola Normale Superiore di Pisa, Pisa, Italy
P. Azzurri, G. Broccolo, R. T. D’Agnolo, L. Foà & F. Ligabue
INFN Sezione di Roma, Roma, Italy
L. Barone, F. Cavallari, D. Del Re, M. Diemoz, C. Fanelli, M. Grassi, E. Longo, P. Meridiani, F. Micheli, S. Nourbakhsh, G. Organtini, F. Pandolfi, R. Paramatti, S. Rahatlou, M. Sigamani & L. Soffi
Università di Roma “La Sapienza”, Roma, Italy
L. Barone, D. Del Re, C. Fanelli, E. Longo, F. Micheli, G. Organtini, F. Pandolfi, S. Rahatlou & L. Soffi
INFN Sezione di Torino, Torino, Italy
N. Amapane, R. Arcidiacono, S. Argiro, M. Arneodo, C. Biino, C. Botta, N. Cartiglia, R. Castello, M. Costa, N. Demaria, A. Graziano, C. Mariotti, S. Maselli, E. Migliore, V. Monaco, M. Musich, M. M. Obertino, N. Pastrone, M. Pelliccioni, A. Potenza, A. Romero, M. Ruspa, R. Sacchi, V. Sola, A. Solano, A. Staiano & A. Vilela Pereira
Università di Torino, Torino, Italy
N. Amapane, S. Argiro, C. Botta, R. Castello, M. Costa, A. Graziano, E. Migliore, V. Monaco, A. Potenza, A. Romero, R. Sacchi, V. Sola & A. Solano
Università del Piemonte Orientale (Novara), Torino, Italy
R. Arcidiacono, M. Arneodo, M. M. Obertino & M. Ruspa
INFN Sezione di Trieste, Trieste, Italy
S. Belforte, F. Cossutti, G. Della Ricca, B. Gobbo, M. Marone, D. Montanino & A. Penzo
Università di Trieste, Trieste, Italy
G. Della Ricca, M. Marone & D. Montanino
Kangwon National University, Chunchon, Korea
S. G. Heo & S. K. Nam
Kyungpook National University, Daegu, Korea
S. Chang, J. Chung, D. H. Kim, G. N. Kim, J. E. Kim, D. J. Kong, H. Park, S. R. Ro & D. C. Son
Institute for Universe and Elementary Particles, Chonnam National University, Kwangju, Korea
J. Y. Kim, Zero J. Kim & S. Song
Konkuk University, Seoul, Korea
H. Y. Jo
Korea University, Seoul, Korea
S. Choi, D. Gyun, B. Hong, M. Jo, H. Kim, T. J. Kim, K. S. Lee, D. H. Moon, S. K. Park, E. Seo & K. S. Sim
University of Seoul, Seoul, Korea
M. Choi, S. Kang, H. Kim, J. H. Kim, C. Park, I. C. Park, S. Park & G. Ryu
Sungkyunkwan University, Suwon, Korea
Y. Cho, Y. Choi, Y. K. Choi, J. Goh, M. S. Kim, B. Lee, J. Lee, S. Lee, H. Seo & I. Yu
Vilnius University, Vilnius, Lithuania
M. J. Bilinskas, I. Grigelionis & M. Janulis
Centro de Investigacion y de Estudios Avanzados del IPN, Mexico City, Mexico
H. Castilla-Valdez, E. De La Cruz-Burelo, I. Heredia-de La Cruz, R. Lopez-Fernandez, R. Magaña Villalba, J. Martínez-Ortega, A. Sánchez-Hernández & L. M. Villasenor-Cendejas
Universidad Iberoamericana, Mexico City, Mexico
S. Carrillo Moreno & F. Vazquez Valencia
Benemerita Universidad Autonoma de Puebla, Puebla, Mexico
H. A. Salazar Ibarguen
Universidad Autónoma de San Luis Potosí, San Luis Potosí, Mexico
E. Casimiro Linares, A. Morelos Pineda & M. A. Reyes-Santos
University of Auckland, Auckland, New Zealand
D. Krofcheck
University of Canterbury, Christchurch, New Zealand
A. J. Bell, P. H. Butler, R. Doesburg, S. Reucroft & H. Silverwood
National Centre for Physics, Quaid-I-Azam University, Islamabad, Pakistan
M. Ahmad, M. I. Asghar, H. R. Hoorani, S. Khalid, W. A. Khan, T. Khurshid, S. Qazi, M. A. Shah & M. Shoaib
Institute of Experimental Physics, Faculty of Physics, University of Warsaw, Warsaw, Poland
G. Brona, M. Cwiok, W. Dominik, K. Doroba, A. Kalinowski, M. Konecki & J. Krolikowski
Soltan Institute for Nuclear Studies, Warsaw, Poland
H. Bialkowska, B. Boimska, T. Frueboes, R. Gokieli, M. Górski, M. Kazana, K. Nawrocki, K. Romanowska-Rybinska, M. Szleper, G. Wrochna & P. Zalewski
Laboratório de Instrumentação e Física Experimental de Partículas, Lisboa, Portugal
N. Almeida, P. Bargassa, A. David, P. Faccioli, P. G. Ferreira Parracho, M. Gallinaro, P. Musella, A. Nayak, J. Pela, P. Q. Ribeiro, J. Seixas, J. Varela & P. Vischia
Joint Institute for Nuclear Research, Dubna, Russia
S. Afanasiev, I. Belotelov, P. Bunin, M. Gavrilenko, I. Golutvin, I. Gorbunov, V. Karjavin, V. Konoplyanikov, G. Kozlov, A. Lanev, P. Moisenz, V. Palichik, V. Perelygin, S. Shmatov, V. Smirnov, A. Volodko & A. Zarubin
Petersburg Nuclear Physics Institute, Gatchina (St Petersburg), Russia
S. Evstyukhin, V. Golovtsov, Y. Ivanov, V. Kim, P. Levchenko, V. Murzin, V. Oreshkin, I. Smirnov, V. Sulimov, L. Uvarov, S. Vavilov, A. Vorobyev & An. Vorobyev
Institute for Nuclear Research, Moscow, Russia
Yu. Andreev, A. Dermenev, S. Gninenko, N. Golubev, M. Kirsanov, N. Krasnikov, V. Matveev, G. Pivovarov, A. Toropin & S. Troitsky
Institute for Theoretical and Experimental Physics, Moscow, Russia
V. Epshteyn, M. Erofeeva, V. Gavrilov, M. Kossov, A. Krokhotin, N. Lychkovskaya, V. Popov, G. Safronov, S. Semenov, V. Stolin, E. Vlasov & A. Zhokin
Moscow State University, Moscow, Russia
A. Belyaev, E. Boos, M. Dubinin, L. Dudko, A. Ershov, L. Khein, O. Kodolova, I. Lokhtin, A. Markina, S. Obraztsov, M. Perfilov, A. Proskuryakov, L. Sarycheva, V. Savrin & A. Snigirev
P.N. Lebedev Physical Institute, Moscow, Russia
V. Andreev, M. Azarkin, I. Dremin, M. Kirakosyan, A. Leonidov, G. Mesyats, S. V. Rusakov & A. Vinogradov
State Research Center of Russian Federation, Institute for High Energy Physics, Protvino, Russia
I. Azhgirey, I. Bayshev, S. Bitioukov, V. Grishin, V. Kachanov, D. Konstantinov, A. Korablev, V. Krychkine, V. Petrov, R. Ryutin, A. Sobol, L. Tourtchanovitch, S. Troshin, N. Tyurin, A. Uzunian & A. Volkov
Faculty of Physics and Vinca Institute of Nuclear Sciences, University of Belgrade, Belgrade, Serbia
P. Adzic, M. Djordjevic, M. Ekmedzic, D. Krpic & J. Milosevic
Centro de Investigaciones Energéticas Medioambientales y Tecnológicas (CIEMAT), Madrid, Spain
M. Aguilar-Benitez, J. Alcaraz Maestre, P. Arce, C. Battilana, E. Calvo, M. Cerrada, M. Chamizo Llatas, N. Colino, B. De La Cruz, A. Delgado Peris, C. Diez Pardos, D. Domínguez Vázquez, C. Fernandez Bedoya, J. P. Fernández Ramos, A. Ferrando, J. Flix, M. C. Fouz, P. Garcia-Abia, O. Gonzalez Lopez, S. Goy Lopez, J. M. Hernandez, M. I. Josa, G. Merino, J. Puerta Pelayo, I. Redondo, L. Romero, J. Santaolalla, M. S. Soares & C. Willmott
Universidad Autónoma de Madrid, Madrid, Spain
C. Albajar, G. Codispoti & J. F. de Trocóniz
Universidad de Oviedo, Oviedo, Spain
J. Cuevas, J. Fernandez Menendez, S. Folgueras, I. Gonzalez Caballero, L. Lloret Iglesias, J. Piedra Gomez & J. M. Vizan Garcia
Instituto de Física de Cantabria (IFCA), CSIC-Universidad de Cantabria, Santander, Spain
J. A. Brochero Cifuentes, I. J. Cabrillo, A. Calderon, S. H. Chuang, J. Duarte Campderros, M. Felcini, M. Fernandez, G. Gomez, J. Gonzalez Sanchez, C. Jorda, P. Lobelle Pardo, A. Lopez Virto, J. Marco, R. Marco, C. Martinez Rivero, F. Matorras, F. J. Munoz Sanchez, T. Rodrigo, A. Y. Rodríguez-Marrero, A. Ruiz-Jimeno, L. Scodellaro, M. Sobron Sanudo, I. Vila & R. Vilar Cortabitarte
CERN, European Organization for Nuclear Research, Geneva, Switzerland
D. Abbaneo, E. Auffray, G. Auzinger, P. Baillon, A. H. Ball, D. Barney, C. Bernet, W. Bialas, G. Bianchi, P. Bloch, A. Bocci, H. Breuker, K. Bunkowski, T. Camporesi, G. Cerminara, T. Christiansen, J. A. Coarasa Perez, B. Curé, D. D’Enterria, A. De Roeck, S. Di Guida, M. Dobson, N. Dupont-Sagorin, A. Elliott-Peisert, B. Frisch, W. Funk, A. Gaddi, G. Georgiou, H. Gerwig, M. Giffels, D. Gigi, K. Gill, D. Giordano, M. Giunta, F. Glege, R. Gomez-Reino Garrido, P. Govoni, S. Gowdy, R. Guida, L. Guiducci, M. Hansen, P. Harris, C. Hartl, J. Harvey, B. Hegner, A. Hinzmann, H. F. Hoffmann, V. Innocente, P. Janot, K. Kaadze, E. Karavakis, K. Kousouris, P. Lecoq, P. Lenzi, C. Lourenço, T. Mäki, M. Malberti, L. Malgeri, M. Mannelli, L. Masetti, G. Mavromanolakis, F. Meijers, S. Mersi, E. Meschi, R. Moser, M. U. Mozer, M. Mulders, E. Nesvold, M. Nguyen, T. Orimoto, L. Orsini, E. Palencia Cortezon, E. Perez, A. Petrilli, A. Pfeiffer, M. Pierini, M. Pimiä, D. Piparo, G. Polese, L. Quertenmont, A. Racz, W. Reece, J. Rodrigues Antunes, G. Rolandi, T. Rommerskirchen, C. Rovelli, M. Rovere, H. Sakulin, F. Santanastasio, C. Schäfer, C. Schwick, I. Segoni, A. Sharma, P. Siegrist, P. Silva, M. Simon, P. Sphicas, D. Spiga, M. Spiropulu, M. Stoye, A. Tsirou, G. I. Veres, P. Vichoudis, H. K. Wöhri, S. D. Worm & W. D. Zeuner
Paul Scherrer Institut, Villigen, Switzerland
W. Bertl, K. Deiters, W. Erdmann, K. Gabathuler, R. Horisberger, Q. Ingram, H. C. Kaestli, S. König, D. Kotlinski, U. Langenegger, F. Meier, D. Renker, T. Rohe & J. Sibille
Institute for Particle Physics, ETH Zurich, Zurich, Switzerland
L. Bäni, P. Bortignon, M. A. Buchmann, B. Casal, N. Chanon, Z. Chen, A. Deisher, G. Dissertori, M. Dittmar, M. Dünser, J. Eugster, K. Freudenreich, C. Grab, P. Lecomte, W. Lustermann, P. Martinez Ruiz del Arbol, N. Mohr, F. Moortgat, C. Nägeli, P. Nef, F. Nessi-Tedaldi, L. Pape, F. Pauss, M. Peruzzi, F. J. Ronga, M. Rossini, L. Sala, A. K. Sanchez, M.-C. Sawley, A. Starodumov, B. Stieger, M. Takahashi, L. Tauscher, A. Thea, K. Theofilatos, D. Treille, C. Urscheler, R. Wallny, H. A. Weber, L. Wehrli & J. Weng
Universität Zürich, Zurich, Switzerland
E. Aguilo, C. Amsler, V. Chiochia, S. De Visscher, C. Favaro, M. Ivova Rikova, B. Millan Mejias, P. Otiougova, P. Robmann, H. Snoek & M. Verzetti
National Central University, Chung-Li, Taiwan
Y. H. Chang, K. H. Chen, C. M. Kuo, S. W. Li, W. Lin, Z. K. Liu, Y. J. Lu, D. Mekterovic, R. Volpe & S. S. Yu
National Taiwan University (NTU), Taipei, Taiwan
P. Bartalini, P. Chang, Y. H. Chang, Y. W. Chang, Y. Chao, K. F. Chen, C. Dietz, U. Grundler, W.-S. Hou, Y. Hsiung, K. Y. Kao, Y. J. Lei, R.-S. Lu, D. Majumder, E. Petrakou, X. Shi, J. G. Shiu, Y. M. Tzeng & M. Wang
Cukurova University, Adana, Turkey
A. Adiguzel, M. N. Bakirci, S. Cerci, C. Dozen, I. Dumanoglu, E. Eskut, S. Girgis, G. Gokbulut, I. Hos, E. E. Kangal, G. Karapinar, A. Kayis Topaksu, G. Onengut, K. Ozdemir, S. Ozturk, A. Polatoz, K. Sogut, D. Sunar Cerci, B. Tali, H. Topakli, D. Uzun, L. N. Vergili & M. Vergili
Middle East Technical University, Physics Department, Ankara, Turkey
I. V. Akin, T. Aliev, B. Bilin, S. Bilmis, M. Deniz, H. Gamsizkan, A. M. Guler, K. Ocalan, A. Ozpineci, M. Serin, R. Sever, U. E. Surat, M. Yalvac, E. Yildirim & M. Zeyrek
Bogazici University, Istanbul, Turkey
M. Deliomeroglu, E. Gülmez, B. Isildak, M. Kaya, O. Kaya, S. Ozkorucuklu & N. Sonmez
National Scientific Center, Kharkov Institute of Physics and Technology, Kharkov, Ukraine
L. Levchuk
University of Bristol, Bristol, United Kingdom
F. Bostock, J. J. Brooke, E. Clement, D. Cussans, H. Flacher, R. Frazier, J. Goldstein, M. Grimes, G. P. Heath, H. F. Heath, L. Kreczko, S. Metson, D. M. Newbold, K. Nirunpong, A. Poll, S. Senkin, V. J. Smith & T. Williams
Rutherford Appleton Laboratory, Didcot, United Kingdom
L. Basso, K. W. Bell, A. Belyaev, C. Brew, R. M. Brown, D. J. A. Cockerill, J. A. Coughlan, K. Harder, S. Harper, J. Jackson, B. W. Kennedy, E. Olaiya, D. Petyt, B. C. Radburn-Smith, C. H. Shepherd-Themistocleous, I. R. Tomalin & W. J. Womersley
Imperial College, London, United Kingdom
R. Bainbridge, G. Ball, R. Beuselinck, O. Buchmuller, D. Colling, N. Cripps, M. Cutajar, P. Dauncey, G. Davies, M. Della Negra, W. Ferguson, J. Fulcher, D. Futyan, A. Gilbert, A. Guneratne Bryer, G. Hall, Z. Hatherell, J. Hays, G. Iles, M. Jarvis, G. Karapostoli, L. Lyons, A.-M. Magnan, J. Marrouche, B. Mathias, R. Nandi, J. Nash, A. Nikitenko, A. Papageorgiou, M. Pesaresi, K. Petridis, M. Pioppi, D. M. Raymond, S. Rogerson, N. Rompotis, A. Rose, M. J. Ryan, C. Seez, A. Sparrow, A. Tapper, S. Tourneur, M. Vazquez Acosta, T. Virdee, S. Wakefield, N. Wardle, D. Wardrope & T. Whyntie
Brunel University, Uxbridge, United Kingdom
M. Barrett, M. Chadwick, J. E. Cole, P. R. Hobson, A. Khan, P. Kyberd, D. Leslie, W. Martin, I. D. Reid, P. Symonds, L. Teodorescu & M. Turner
Baylor University, Waco, USA
K. Hatakeyama, H. Liu & T. Scarborough
The University of Alabama, Tuscaloosa, USA
C. Henderson
Boston University, Boston, USA
A. Avetisyan, T. Bose, E. Carrera Jarrin, C. Fantasia, A. Heister, J. St. John, P. Lawson, D. Lazic, J. Rohlf, D. Sperka & L. Sulak
Brown University, Providence, USA
S. Bhattacharya, D. Cutts, A. Ferapontov, U. Heintz, S. Jabeen, G. Kukartsev, G. Landsberg, M. Luk, M. Narain, D. Nguyen, M. Segala, T. Sinthuprasith, T. Speer & K. V. Tsang
University of California, Davis, Davis, USA
R. Breedon, G. Breto, M. Calderon De La Barca Sanchez, M. Caulfield, S. Chauhan, M. Chertok, J. Conway, R. Conway, P. T. Cox, J. Dolen, R. Erbacher, M. Gardner, R. Houtz, W. Ko, A. Kopecky, R. Lander, O. Mall, T. Miceli, R. Nelson, D. Pellett, J. Robles, B. Rutherford, M. Searle, J. Smith, M. Squires, M. Tripathi & R. Vasquez Sierra
University of California, Los Angeles, Los Angeles, USA
V. Andreev, K. Arisaka, D. Cline, R. Cousins, J. Duris, S. Erhan, P. Everaerts, C. Farrell, J. Hauser, M. Ignatenko, C. Jarvis, C. Plager, G. Rakness, P. Schlein, J. Tucker, V. Valuev & M. Weber
University of California, Riverside, Riverside, USA
J. Babb, R. Clare, J. Ellison, J. W. Gary, F. Giordano, G. Hanson, G. Y. Jeng, H. Liu, O. R. Long, A. Luthra, H. Nguyen, S. Paramesvaran, J. Sturdy, S. Sumowidagdo, R. Wilken & S. Wimpenny
University of California, San Diego, La Jolla, USA
W. Andrews, J. G. Branson, G. B. Cerati, S. Cittolin, D. Evans, F. Golf, A. Holzner, R. Kelley, M. Lebourgeois, J. Letts, I. Macneill, B. Mangano, S. Padhi, C. Palmer, G. Petrucciani, H. Pi, M. Pieri, R. Ranieri, M. Sani, I. Sfiligoi, V. Sharma, S. Simon, E. Sudano, M. Tadel, Y. Tu, A. Vartak, S. Wasserbaech, F. Würthwein, A. Yagil & J. Yoo
University of California, Santa Barbara, Santa Barbara, USA
D. Barge, R. Bellan, C. Campagnari, M. D’Alfonso, T. Danielson, K. Flowers, P. Geffert, J. Incandela, C. Justus, P. Kalavase, S. A. Koay, D. Kovalskyi, V. Krutelyov, S. Lowette, N. Mccoll, V. Pavlunin, F. Rebassoo, J. Ribnik, J. Richman, R. Rossin, D. Stuart, W. To, J. R. Vlimant & C. West
California Institute of Technology, Pasadena, USA
A. Apresyan, A. Bornheim, J. Bunn, Y. Chen, E. Di Marco, J. Duarte, M. Gataullin, Y. Ma, A. Mott, H. B. Newman, C. Rogan, V. Timciuc, P. Traczyk, J. Veverka, R. Wilkinson, Y. Yang & R. Y. Zhu
Carnegie Mellon University, Pittsburgh, USA
B. Akgun, R. Carroll, T. Ferguson, Y. Iiyama, D. W. Jang, S. Y. Jun, Y. F. Liu, M. Paulini, J. Russ, H. Vogel & I. Vorobiev
University of Colorado at Boulder, Boulder, USA
J. P. Cumalat, M. E. Dinardo, B. R. Drell, C. J. Edelmaier, W. T. Ford, A. Gaz, B. Heyburn, E. Luiggi Lopez, U. Nauenberg, J. G. Smith, K. Stenson, K. A. Ulmer, S. R. Wagner & S. L. Zang
Cornell University, Ithaca, USA
L. Agostino, J. Alexander, A. Chatterjee, N. Eggert, L. K. Gibbons, B. Heltsley, W. Hopkins, A. Khukhunaishvili, B. Kreis, N. Mirman, G. Nicolas Kaufman, J. R. Patterson, A. Ryd, E. Salvati, W. Sun, W. D. Teo, J. Thom, J. Thompson, J. Vaughan, Y. Weng, L. Winstrom & P. Wittich
Fairfield University, Fairfield, USA
A. Biselli & D. Winn
Fermi National Accelerator Laboratory, Batavia, USA
S. Abdullin, M. Albrow, J. Anderson, G. Apollinari, M. Atac, J. A. Bakken, L. A. T. Bauerdick, A. Beretvas, J. Berryhill, P. C. Bhat, I. Bloch, K. Burkett, J. N. Butler, V. Chetluru, H. W. K. Cheung, F. Chlebana, S. Cihangir, W. Cooper, D. P. Eartly, V. D. Elvira, S. Esen, I. Fisk, J. Freeman, Y. Gao, E. Gottschalk, D. Green, O. Gutsche, J. Hanlon, R. M. Harris, J. Hirschauer, B. Hooberman, H. Jensen, S. Jindariani, M. Johnson, U. Joshi, B. Klima, S. Kunori, S. Kwan, C. Leonidopoulos, D. Lincoln, R. Lipton, J. Lykken, K. Maeshima, J. M. Marraffino, S. Maruyama, D. Mason, P. McBride, T. Miao, K. Mishra, S. Mrenna, Y. Musienko, C. Newman-Holmes, V. O’Dell, J. Pivarski, R. Pordes, O. Prokofyev, T. Schwarz, E. Sexton-Kennedy, S. Sharma, W. J. Spalding, L. Spiegel, P. Tan, L. Taylor, S. Tkaczyk, L. Uplegger, E. W. Vaandering, R. Vidal, J. Whitmore, W. Wu, F. Yang, F. Yumiceva & J. C. Yun
University of Florida, Gainesville, USA
D. Acosta, P. Avery, D. Bourilkov, M. Chen, S. Das, M. De Gruttola, G. P. Di Giovanni, D. Dobur, A. Drozdetskiy, R. D. Field, M. Fisher, Y. Fu, I. K. Furic, J. Gartner, S. Goldberg, J. Hugon, B. Kim, J. Konigsberg, A. Korytov, A. Kropivnitskaya, T. Kypreos, J. F. Low, K. Matchev, P. Milenovic, G. Mitselmakher, L. Muniz, R. Remington, A. Rinkevicius, M. Schmitt, B. Scurlock, P. Sellers, N. Skhirtladze, M. Snowball, D. Wang, J. Yelton & M. Zakaria
Florida International University, Miami, USA
V. Gaultney, L. M. Lebolo, S. Linn, P. Markowitz, G. Martinez & J. L. Rodriguez
Florida State University, Tallahassee, USA
T. Adams, A. Askew, J. Bochenek, J. Chen, B. Diamond, S. V. Gleyzer, J. Haas, S. Hagopian, V. Hagopian, M. Jenkins, K. F. Johnson, H. Prosper, S. Sekmen, V. Veeraraghavan & M. Weinberg
Florida Institute of Technology, Melbourne, USA
M. M. Baarmand, B. Dorney, M. Hohlmann, H. Kalakhety & I. Vodopiyanov
University of Illinois at Chicago (UIC), Chicago, USA
M. R. Adams, I. M. Anghel, L. Apanasevich, Y. Bai, V. E. Bazterra, R. R. Betts, J. Callner, R. Cavanaugh, C. Dragoiu, L. Gauthier, C. E. Gerber, D. J. Hofman, S. Khalatyan, G. J. Kunde, F. Lacroix, M. Malek, C. O’Brien, C. Silkworth, C. Silvestre, D. Strom & N. Varelas
The University of Iowa, Iowa City, USA
U. Akgun, E. A. Albayrak, B. Bilki, W. Clarida, F. Duru, S. Griffiths, C. K. Lae, E. McCliment, J.-P. Merlo, H. Mermerkaya, A. Mestvirishvili, A. Moeller, J. Nachtman, C. R. Newsom, E. Norbeck, J. Olson, Y. Onel, F. Ozok, S. Sen, E. Tiras, J. Wetzel, T. Yetkin & K. Yi
Johns Hopkins University, Baltimore, USA
B. A. Barnett, B. Blumenfeld, S. Bolognesi, A. Bonato, D. Fehling, G. Giurgiu, A. V. Gritsan, Z. J. Guo, G. Hu, P. Maksimovic, S. Rappoccio, M. Swartz, N. V. Tran & A. Whitbeck
The University of Kansas, Lawrence, USA
P. Baringer, A. Bean, G. Benelli, O. Grachov, R. P. Kenny Iii, M. Murray, D. Noonan, S. Sanders, R. Stringer, G. Tinti, J. S. Wood & V. Zhukova
Kansas State University, Manhattan, USA
A. F. Barfuss, T. Bolton, I. Chakaberia, A. Ivanov, S. Khalil, M. Makouski, Y. Maravin, S. Shrestha & I. Svintradze
Lawrence Livermore National Laboratory, Livermore, USA
J. Gronberg, D. Lange & D. Wright
University of Maryland, College Park, USA
A. Baden, M. Boutemeur, B. Calvert, S. C. Eno, J. A. Gomez, N. J. Hadley, R. G. Kellogg, M. Kirn, T. Kolberg, Y. Lu, M. Marionneau, A. C. Mignerey, A. Peterman, K. Rossato, P. Rumerio, A. Skuja, J. Temple, M. B. Tonjes, S. C. Tonwar & E. Twedt
Massachusetts Institute of Technology, Cambridge, USA
B. Alver, G. Bauer, J. Bendavid, W. Busza, E. Butz, I. A. Cali, M. Chan, V. Dutta, G. Gomez Ceballos, M. Goncharov, K. A. Hahn, Y. Kim, M. Klute, Y.-J. Lee, W. Li, P. D. Luckey, T. Ma, S. Nahn, C. Paus, D. Ralph, C. Roland, G. Roland, M. Rudolph, G. S. F. Stephans, F. Stöckli, K. Sumorok, K. Sung, D. Velicanu, E. A. Wenger, R. Wolf, B. Wyslouch, S. Xie, M. Yang, Y. Yilmaz, A. S. Yoon & M. Zanetti
University of Minnesota, Minneapolis, USA
S. I. Cooper, P. Cushman, B. Dahmes, A. De Benedetti, G. Franzoni, A. Gude, J. Haupt, S. C. Kao, K. Klapoetke, Y. Kubota, J. Mans, N. Pastika, V. Rekovic, R. Rusack, M. Sasseville, A. Singovsky, N. Tambe & J. Turkewitz
University of Mississippi, University, USA
L. M. Cremaldi, R. Godang, R. Kroeger, L. Perera, R. Rahmat, D. A. Sanders & D. Summers
University of Nebraska-Lincoln, Lincoln, USA
E. Avdeeva, K. Bloom, S. Bose, J. Butt, D. R. Claes, A. Dominguez, M. Eads, P. Jindal, J. Keller, I. Kravchenko, J. Lazo-Flores, H. Malbouisson, S. Malik & G. R. Snow
State University of New York at Buffalo, Buffalo, USA
U. Baur, A. Godshalk, I. Iashvili, S. Jain, A. Kharchilava, A. Kumar, S. P. Shipkowski, K. Smith & Z. Wan
Northeastern University, Boston, USA
G. Alverson, E. Barberis, D. Baumgartel, M. Chasco, D. Trocino, D. Wood & J. Zhang
Northwestern University, Evanston, USA
A. Anastassov, A. Kubik, N. Mucia, N. Odell, R. A. Ofierzynski, B. Pollack, A. Pozdnyakov, M. Schmitt, S. Stoynev, M. Velasco & S. Won
University of Notre Dame, Notre Dame, USA
L. Antonelli, D. Berry, A. Brinkerhoff, M. Hildreth, C. Jessop, D. J. Karmgard, J. Kolb, K. Lannon, W. Luo, S. Lynch, N. Marinelli, D. M. Morse, T. Pearson, R. Ruchti, J. Slaunwhite, N. Valls, M. Wayne, M. Wolf & J. Ziegler
The Ohio State University, Columbus, USA
B. Bylsma, L. S. Durkin, C. Hill, P. Killewald, K. Kotov, T. Y. Ling, D. Puigh, M. Rodenburg, C. Vuosalo & G. Williams
Princeton University, Princeton, USA
N. Adam, E. Berry, P. Elmer, D. Gerbaudo, V. Halyo, P. Hebda, J. Hegeman, A. Hunt, E. Laird, D. Lopes Pegna, P. Lujan, D. Marlow, T. Medvedeva, M. Mooney, J. Olsen, P. Piroué, X. Quan, A. Raval, H. Saka, D. Stickland, C. Tully, J. S. Werner & A. Zuranski
University of Puerto Rico, Mayaguez, USA
J. G. Acosta, X. T. Huang, A. Lopez, H. Mendez, S. Oliveros, J. E. Ramirez Vargas & A. Zatserklyaniy
Purdue University, West Lafayette, USA
E. Alagoz, V. E. Barnes, D. Benedetti, G. Bolla, D. Bortoletto, M. De Mattia, A. Everett, L. Gutay, Z. Hu, M. Jones, O. Koybasi, M. Kress, A. T. Laasanen, N. Leonardo, V. Maroussov, P. Merkel, D. H. Miller, N. Neumeister, I. Shipsey, D. Silvers, A. Svyatkovskiy, M. Vidal Marono, H. D. Yoo, J. Zablocki & Y. Zheng
Purdue University Calumet, Hammond, USA
S. Guragain & N. Parashar
Rice University, Houston, USA
A. Adair, C. Boulahouache, V. Cuplov, K. M. Ecklund, F. J. M. Geurts, B. P. Padley, R. Redjimi, J. Roberts & J. Zabel
University of Rochester, Rochester, USA
B. Betchart, A. Bodek, Y. S. Chung, R. Covarelli, P. de Barbaro, R. Demina, Y. Eshaq, A. Garcia-Bellido, P. Goldenzweig, Y. Gotra, J. Han, A. Harel, D. C. Miner, G. Petrillo, W. Sakumoto, D. Vishnevskiy & M. Zielinski
The Rockefeller University, New York, USA
A. Bhatti, R. Ciesielski, L. Demortier, K. Goulianos, G. Lungu, S. Malik & C. Mesropian
Rutgers, the State University of New Jersey, Piscataway, USA
S. Arora, O. Atramentov, A. Barker, J. P. Chou, C. Contreras-Campana, E. Contreras-Campana, D. Duggan, D. Ferencek, Y. Gershtein, R. Gray, E. Halkiadakis, D. Hidas, D. Hits, A. Lath, S. Panwalkar, M. Park, R. Patel, A. Richards, K. Rose, S. Salur, S. Schnetzer, C. Seitz, S. Somalwar, R. Stone & S. Thomas
University of Tennessee, Knoxville, USA
G. Cerizza, M. Hollingsworth, S. Spanier, Z. C. Yang & A. York
Texas A&M University, College Station, USA
R. Eusebi, W. Flanagan, J. Gilmore, T. Kamon, V. Khotilovich, R. Montalvo, I. Osipenkov, Y. Pakhotin, A. Perloff, J. Roe, A. Safonov, T. Sakuma, S. Sengupta, I. Suarez, A. Tatarinov & D. Toback
Texas Tech University, Lubbock, USA
N. Akchurin, C. Bardak, J. Damgov, P. R. Dudero, C. Jeong, K. Kovitanggoon, S. W. Lee, T. Libeiro, P. Mane, Y. Roh, A. Sill, I. Volobouev & R. Wigmans
Vanderbilt University, Nashville, USA
E. Appelt, E. Brownson, D. Engh, C. Florez, W. Gabella, A. Gurrola, M. Issah, W. Johns, P. Kurt, C. Maguire, A. Melo, P. Sheldon, B. Snook, S. Tuo & J. Velkovska
University of Virginia, Charlottesville, USA
M. W. Arenton, M. Balazs, S. Boutle, S. Conetti, B. Cox, B. Francis, S. Goadhouse, J. Goodell, R. Hirosky, A. Ledovskoy, C. Lin, C. Neu, J. Wood & R. Yohay
Wayne State University, Detroit, USA
S. Gollapinni, R. Harr, P. E. Karchin, C. Kottachchi Kankanamge Don, P. Lamichhane, M. Mattson, C. Milstène & A. Sakharov
University of Wisconsin, Madison, USA
M. Anderson, M. Bachtis, D. Belknap, J. N. Bellinger, J. Bernardini, L. Borrello, D. Carlsmith, M. Cepeda, S. Dasu, J. Efron, E. Friis, L. Gray, K. S. Grogg, M. Grothe, R. Hall-Wilton, M. Herndon, A. Hervé, P. Klabbers, J. Klukas, A. Lanaro, C. Lazaridis, J. Leonard, R. Loveless, A. Mohapatra, I. Ojalvo, G. A. Pierro, I. Ross, A. Savin, W. H. Smith & J. Swanson
- S. Chatrchyan
You can also search for this author in PubMed Google Scholar
- V. Khachatryan
You can also search for this author in PubMed Google Scholar
- A. M. Sirunyan
You can also search for this author in PubMed Google Scholar
- A. Tumasyan
You can also search for this author in PubMed Google Scholar
- W. Adam
You can also search for this author in PubMed Google Scholar
- T. Bergauer
You can also search for this author in PubMed Google Scholar
- M. Dragicevic
You can also search for this author in PubMed Google Scholar
- J. Erö
You can also search for this author in PubMed Google Scholar
- C. Fabjan
You can also search for this author in PubMed Google Scholar
- M. Friedl
You can also search for this author in PubMed Google Scholar
- R. Frühwirth
You can also search for this author in PubMed Google Scholar
- V. M. Ghete
You can also search for this author in PubMed Google Scholar
- J. Hammer
You can also search for this author in PubMed Google Scholar
- M. Hoch
You can also search for this author in PubMed Google Scholar
- N. Hörmann
You can also search for this author in PubMed Google Scholar
- J. Hrubec
You can also search for this author in PubMed Google Scholar
- M. Jeitler
You can also search for this author in PubMed Google Scholar
- W. Kiesenhofer
You can also search for this author in PubMed Google Scholar
- M. Krammer
You can also search for this author in PubMed Google Scholar
- D. Liko
You can also search for this author in PubMed Google Scholar
- I. Mikulec
You can also search for this author in PubMed Google Scholar
- M. Pernicka
You can also search for this author in PubMed Google Scholar
- B. Rahbaran
You can also search for this author in PubMed Google Scholar
- C. Rohringer
You can also search for this author in PubMed Google Scholar
- H. Rohringer
You can also search for this author in PubMed Google Scholar
- R. Schöfbeck
You can also search for this author in PubMed Google Scholar
- J. Strauss
You can also search for this author in PubMed Google Scholar
- A. Taurok
You can also search for this author in PubMed Google Scholar
- F. Teischinger
You can also search for this author in PubMed Google Scholar
- P. Wagner
You can also search for this author in PubMed Google Scholar
- W. Waltenberger
You can also search for this author in PubMed Google Scholar
- G. Walzel
You can also search for this author in PubMed Google Scholar
- E. Widl
You can also search for this author in PubMed Google Scholar
- C.-E. Wulz
You can also search for this author in PubMed Google Scholar
- V. Mossolov
You can also search for this author in PubMed Google Scholar
- N. Shumeiko
You can also search for this author in PubMed Google Scholar
- J. Suarez Gonzalez
You can also search for this author in PubMed Google Scholar
- S. Bansal
You can also search for this author in PubMed Google Scholar
- L. Benucci
You can also search for this author in PubMed Google Scholar
- T. Cornelis
You can also search for this author in PubMed Google Scholar
- E. A. De Wolf
You can also search for this author in PubMed Google Scholar
- X. Janssen
You can also search for this author in PubMed Google Scholar
- S. Luyckx
You can also search for this author in PubMed Google Scholar
- T. Maes
You can also search for this author in PubMed Google Scholar
- L. Mucibello
You can also search for this author in PubMed Google Scholar
- S. Ochesanu
You can also search for this author in PubMed Google Scholar
- B. Roland
You can also search for this author in PubMed Google Scholar
- R. Rougny
You can also search for this author in PubMed Google Scholar
- M. Selvaggi
You can also search for this author in PubMed Google Scholar
- H. Van Haevermaet
You can also search for this author in PubMed Google Scholar
- P. Van Mechelen
You can also search for this author in PubMed Google Scholar
- N. Van Remortel
You can also search for this author in PubMed Google Scholar
- A. Van Spilbeeck
You can also search for this author in PubMed Google Scholar
- F. Blekman
You can also search for this author in PubMed Google Scholar
- S. Blyweert
You can also search for this author in PubMed Google Scholar
- J. D’Hondt
You can also search for this author in PubMed Google Scholar
- R. Gonzalez Suarez
You can also search for this author in PubMed Google Scholar
- A. Kalogeropoulos
You can also search for this author in PubMed Google Scholar
- M. Maes
You can also search for this author in PubMed Google Scholar
- A. Olbrechts
You can also search for this author in PubMed Google Scholar
- W. Van Doninck
You can also search for this author in PubMed Google Scholar
- P. Van Mulders
You can also search for this author in PubMed Google Scholar
- G. P. Van Onsem
You can also search for this author in PubMed Google Scholar
- I. Villella
You can also search for this author in PubMed Google Scholar
- O. Charaf
You can also search for this author in PubMed Google Scholar
- B. Clerbaux
You can also search for this author in PubMed Google Scholar
- G. De Lentdecker
You can also search for this author in PubMed Google Scholar
- V. Dero
You can also search for this author in PubMed Google Scholar
- A. P. R. Gay
You can also search for this author in PubMed Google Scholar
- G. H. Hammad
You can also search for this author in PubMed Google Scholar
- T. Hreus
You can also search for this author in PubMed Google Scholar
- A. Léonard
You can also search for this author in PubMed Google Scholar
- P. E. Marage
You can also search for this author in PubMed Google Scholar
- L. Thomas
You can also search for this author in PubMed Google Scholar
- C. Vander Velde
You can also search for this author in PubMed Google Scholar
- P. Vanlaer
You can also search for this author in PubMed Google Scholar
- J. Wickens
You can also search for this author in PubMed Google Scholar
- V. Adler
You can also search for this author in PubMed Google Scholar
- K. Beernaert
You can also search for this author in PubMed Google Scholar
- A. Cimmino
You can also search for this author in PubMed Google Scholar
- S. Costantini
You can also search for this author in PubMed Google Scholar
- G. Garcia
You can also search for this author in PubMed Google Scholar
- M. Grunewald
You can also search for this author in PubMed Google Scholar
- B. Klein
You can also search for this author in PubMed Google Scholar
- J. Lellouch
You can also search for this author in PubMed Google Scholar
- A. Marinov
You can also search for this author in PubMed Google Scholar
- J. Mccartin
You can also search for this author in PubMed Google Scholar
- A. A. Ocampo Rios
You can also search for this author in PubMed Google Scholar
- D. Ryckbosch
You can also search for this author in PubMed Google Scholar
- N. Strobbe
You can also search for this author in PubMed Google Scholar
- F. Thyssen
You can also search for this author in PubMed Google Scholar
- M. Tytgat
You can also search for this author in PubMed Google Scholar
- L. Vanelderen
You can also search for this author in PubMed Google Scholar
- P. Verwilligen
You can also search for this author in PubMed Google Scholar
- S. Walsh
You can also search for this author in PubMed Google Scholar
- E. Yazgan
You can also search for this author in PubMed Google Scholar
- N. Zaganidis
You can also search for this author in PubMed Google Scholar
- S. Basegmez
You can also search for this author in PubMed Google Scholar
- G. Bruno
You can also search for this author in PubMed Google Scholar
- L. Ceard
You can also search for this author in PubMed Google Scholar
- J. De Favereau De Jeneret
You can also search for this author in PubMed Google Scholar
- C. Delaere
You can also search for this author in PubMed Google Scholar
- T. du Pree
You can also search for this author in PubMed Google Scholar
- D. Favart
You can also search for this author in PubMed Google Scholar
- L. Forthomme
You can also search for this author in PubMed Google Scholar
- A. Giammanco
You can also search for this author in PubMed Google Scholar
- G. Grégoire
You can also search for this author in PubMed Google Scholar
- J. Hollar
You can also search for this author in PubMed Google Scholar
- V. Lemaitre
You can also search for this author in PubMed Google Scholar
- J. Liao
You can also search for this author in PubMed Google Scholar
- O. Militaru
You can also search for this author in PubMed Google Scholar
- C. Nuttens
You can also search for this author in PubMed Google Scholar
- D. Pagano
You can also search for this author in PubMed Google Scholar
- A. Pin
You can also search for this author in PubMed Google Scholar
- K. Piotrzkowski
You can also search for this author in PubMed Google Scholar
- N. Schul
You can also search for this author in PubMed Google Scholar
- N. Beliy
You can also search for this author in PubMed Google Scholar
- T. Caebergs
You can also search for this author in PubMed Google Scholar
- E. Daubie
You can also search for this author in PubMed Google Scholar
- G. A. Alves
You can also search for this author in PubMed Google Scholar
- M. Correa Martins Junior
You can also search for this author in PubMed Google Scholar
- D. De Jesus Damiao
You can also search for this author in PubMed Google Scholar
- T. Martins
You can also search for this author in PubMed Google Scholar
- M. E. Pol
You can also search for this author in PubMed Google Scholar
- M. H. G. Souza
You can also search for this author in PubMed Google Scholar
- W. L. Aldá Júnior
You can also search for this author in PubMed Google Scholar
- W. Carvalho
You can also search for this author in PubMed Google Scholar
- A. Custódio
You can also search for this author in PubMed Google Scholar
- E. M. Da Costa
You can also search for this author in PubMed Google Scholar
- C. De Oliveira Martins
You can also search for this author in PubMed Google Scholar
- S. Fonseca De Souza
You can also search for this author in PubMed Google Scholar
- D. Matos Figueiredo
You can also search for this author in PubMed Google Scholar
- L. Mundim
You can also search for this author in PubMed Google Scholar
- H. Nogima
You can also search for this author in PubMed Google Scholar
- V. Oguri
You can also search for this author in PubMed Google Scholar
- W. L. Prado Da Silva
You can also search for this author in PubMed Google Scholar
- A. Santoro
You can also search for this author in PubMed Google Scholar
- S. M. Silva Do Amaral
You can also search for this author in PubMed Google Scholar
- L. Soares Jorge
You can also search for this author in PubMed Google Scholar
- A. Sznajder
You can also search for this author in PubMed Google Scholar
- T. S. Anjos
You can also search for this author in PubMed Google Scholar
- C. A. Bernardes
You can also search for this author in PubMed Google Scholar
- F. A. Dias
You can also search for this author in PubMed Google Scholar
- T. R. Fernandez Perez Tomei
You can also search for this author in PubMed Google Scholar
- E. M. Gregores
You can also search for this author in PubMed Google Scholar
- C. Lagana
You can also search for this author in PubMed Google Scholar
- F. Marinho
You can also search for this author in PubMed Google Scholar
- P. G. Mercadante
You can also search for this author in PubMed Google Scholar
- S. F. Novaes
You can also search for this author in PubMed Google Scholar
- Sandra S. Padula
You can also search for this author in PubMed Google Scholar
- V. Genchev
You can also search for this author in PubMed Google Scholar
- P. Iaydjiev
You can also search for this author in PubMed Google Scholar
- S. Piperov
You can also search for this author in PubMed Google Scholar
- M. Rodozov
You can also search for this author in PubMed Google Scholar
- S. Stoykova
You can also search for this author in PubMed Google Scholar
- G. Sultanov
You can also search for this author in PubMed Google Scholar
- V. Tcholakov
You can also search for this author in PubMed Google Scholar
- R. Trayanov
You can also search for this author in PubMed Google Scholar
- M. Vutova
You can also search for this author in PubMed Google Scholar
- A. Dimitrov
You can also search for this author in PubMed Google Scholar
- R. Hadjiiska
You can also search for this author in PubMed Google Scholar
- A. Karadzhinova
You can also search for this author in PubMed Google Scholar
- V. Kozhuharov
You can also search for this author in PubMed Google Scholar
- L. Litov
You can also search for this author in PubMed Google Scholar
- B. Pavlov
You can also search for this author in PubMed Google Scholar
- P. Petkov
You can also search for this author in PubMed Google Scholar
- J. G. Bian
You can also search for this author in PubMed Google Scholar
- G. M. Chen
You can also search for this author in PubMed Google Scholar
- H. S. Chen
You can also search for this author in PubMed Google Scholar
- C. H. Jiang
You can also search for this author in PubMed Google Scholar
- D. Liang
You can also search for this author in PubMed Google Scholar
- S. Liang
You can also search for this author in PubMed Google Scholar
- X. Meng
You can also search for this author in PubMed Google Scholar
- J. Tao
You can also search for this author in PubMed Google Scholar
- J. Wang
You can also search for this author in PubMed Google Scholar
- J. Wang
You can also search for this author in PubMed Google Scholar
- X. Wang
You can also search for this author in PubMed Google Scholar
- Z. Wang
You can also search for this author in PubMed Google Scholar
- H. Xiao
You can also search for this author in PubMed Google Scholar
- M. Xu
You can also search for this author in PubMed Google Scholar
- J. Zang
You can also search for this author in PubMed Google Scholar
- Z. Zhang
You can also search for this author in PubMed Google Scholar
- C. Asawatangtrakuldee
You can also search for this author in PubMed Google Scholar
- Y. Ban
You can also search for this author in PubMed Google Scholar
- S. Guo
You can also search for this author in PubMed Google Scholar
- Y. Guo
You can also search for this author in PubMed Google Scholar
- W. Li
You can also search for this author in PubMed Google Scholar
- S. Liu
You can also search for this author in PubMed Google Scholar
- Y. Mao
You can also search for this author in PubMed Google Scholar
- S. J. Qian
You can also search for this author in PubMed Google Scholar
- H. Teng
You can also search for this author in PubMed Google Scholar
- S. Wang
You can also search for this author in PubMed Google Scholar
- B. Zhu
You can also search for this author in PubMed Google Scholar
- W. Zou
You can also search for this author in PubMed Google Scholar
- A. Cabrera
You can also search for this author in PubMed Google Scholar
- B. Gomez Moreno
You can also search for this author in PubMed Google Scholar
- A. F. Osorio Oliveros
You can also search for this author in PubMed Google Scholar
- J. C. Sanabria
You can also search for this author in PubMed Google Scholar
- N. Godinovic
You can also search for this author in PubMed Google Scholar
- D. Lelas
You can also search for this author in PubMed Google Scholar
- R. Plestina
You can also search for this author in PubMed Google Scholar
- D. Polic
You can also search for this author in PubMed Google Scholar
- I. Puljak
You can also search for this author in PubMed Google Scholar
- Z. Antunovic
You can also search for this author in PubMed Google Scholar
- M. Dzelalija
You can also search for this author in PubMed Google Scholar
- M. Kovac
You can also search for this author in PubMed Google Scholar
- V. Brigljevic
You can also search for this author in PubMed Google Scholar
- S. Duric
You can also search for this author in PubMed Google Scholar
- K. Kadija
You can also search for this author in PubMed Google Scholar
- J. Luetic
You can also search for this author in PubMed Google Scholar
- S. Morovic
You can also search for this author in PubMed Google Scholar
- A. Attikis
You can also search for this author in PubMed Google Scholar
- M. Galanti
You can also search for this author in PubMed Google Scholar
- J. Mousa
You can also search for this author in PubMed Google Scholar
- C. Nicolaou
You can also search for this author in PubMed Google Scholar
- F. Ptochos
You can also search for this author in PubMed Google Scholar
- P. A. Razis
You can also search for this author in PubMed Google Scholar
- M. Finger
You can also search for this author in PubMed Google Scholar
- M. Finger Jr.
You can also search for this author in PubMed Google Scholar
- Y. Assran
You can also search for this author in PubMed Google Scholar
- A. Ellithi Kamel
You can also search for this author in PubMed Google Scholar
- S. Khalil
You can also search for this author in PubMed Google Scholar
- M. A. Mahmoud
You can also search for this author in PubMed Google Scholar
- A. Radi
You can also search for this author in PubMed Google Scholar
- A. Hektor
You can also search for this author in PubMed Google Scholar
- M. Kadastik
You can also search for this author in PubMed Google Scholar
- M. Müntel
You can also search for this author in PubMed Google Scholar
- M. Raidal
You can also search for this author in PubMed Google Scholar
- L. Rebane
You can also search for this author in PubMed Google Scholar
- A. Tiko
You can also search for this author in PubMed Google Scholar
- V. Azzolini
You can also search for this author in PubMed Google Scholar
- P. Eerola
You can also search for this author in PubMed Google Scholar
- G. Fedi
You can also search for this author in PubMed Google Scholar
- M. Voutilainen
You can also search for this author in PubMed Google Scholar
- S. Czellar
You can also search for this author in PubMed Google Scholar
- J. Härkönen
You can also search for this author in PubMed Google Scholar
- A. Heikkinen
You can also search for this author in PubMed Google Scholar
- V. Karimäki
You can also search for this author in PubMed Google Scholar
- R. Kinnunen
You can also search for this author in PubMed Google Scholar
- M. J. Kortelainen
You can also search for this author in PubMed Google Scholar
- T. Lampén
You can also search for this author in PubMed Google Scholar
- K. Lassila-Perini
You can also search for this author in PubMed Google Scholar
- S. Lehti
You can also search for this author in PubMed Google Scholar
- T. Lindén
You can also search for this author in PubMed Google Scholar
- P. Luukka
You can also search for this author in PubMed Google Scholar
- T. Mäenpää
You can also search for this author in PubMed Google Scholar
- T. Peltola
You can also search for this author in PubMed Google Scholar
- E. Tuominen
You can also search for this author in PubMed Google Scholar
- J. Tuominiemi
You can also search for this author in PubMed Google Scholar
- E. Tuovinen
You can also search for this author in PubMed Google Scholar
- D. Ungaro
You can also search for this author in PubMed Google Scholar
- L. Wendland
You can also search for this author in PubMed Google Scholar
- K. Banzuzi
You can also search for this author in PubMed Google Scholar
- A. Korpela
You can also search for this author in PubMed Google Scholar
- T. Tuuva
You can also search for this author in PubMed Google Scholar
- D. Sillou
You can also search for this author in PubMed Google Scholar
- M. Besancon
You can also search for this author in PubMed Google Scholar
- S. Choudhury
You can also search for this author in PubMed Google Scholar
- M. Dejardin
You can also search for this author in PubMed Google Scholar
- D. Denegri
You can also search for this author in PubMed Google Scholar
- B. Fabbro
You can also search for this author in PubMed Google Scholar
- J. L. Faure
You can also search for this author in PubMed Google Scholar
- F. Ferri
You can also search for this author in PubMed Google Scholar
- S. Ganjour
You can also search for this author in PubMed Google Scholar
- A. Givernaud
You can also search for this author in PubMed Google Scholar
- P. Gras
You can also search for this author in PubMed Google Scholar
- G. Hamel de Monchenault
You can also search for this author in PubMed Google Scholar
- P. Jarry
You can also search for this author in PubMed Google Scholar
- E. Locci
You can also search for this author in PubMed Google Scholar
- J. Malcles
You can also search for this author in PubMed Google Scholar
- L. Millischer
You can also search for this author in PubMed Google Scholar
- J. Rander
You can also search for this author in PubMed Google Scholar
- A. Rosowsky
You can also search for this author in PubMed Google Scholar
- I. Shreyber
You can also search for this author in PubMed Google Scholar
- M. Titov
You can also search for this author in PubMed Google Scholar
- S. Baffioni
You can also search for this author in PubMed Google Scholar
- F. Beaudette
You can also search for this author in PubMed Google Scholar
- L. Benhabib
You can also search for this author in PubMed Google Scholar
- L. Bianchini
You can also search for this author in PubMed Google Scholar
- M. Bluj
You can also search for this author in PubMed Google Scholar
- C. Broutin
You can also search for this author in PubMed Google Scholar
- P. Busson
You can also search for this author in PubMed Google Scholar
- C. Charlot
You can also search for this author in PubMed Google Scholar
- N. Daci
You can also search for this author in PubMed Google Scholar
- T. Dahms
You can also search for this author in PubMed Google Scholar
- L. Dobrzynski
You can also search for this author in PubMed Google Scholar
- S. Elgammal
You can also search for this author in PubMed Google Scholar
- R. Granier de Cassagnac
You can also search for this author in PubMed Google Scholar
- M. Haguenauer
You can also search for this author in PubMed Google Scholar
- P. Miné
You can also search for this author in PubMed Google Scholar
- C. Mironov
You can also search for this author in PubMed Google Scholar
- C. Ochando
You can also search for this author in PubMed Google Scholar
- P. Paganini
You can also search for this author in PubMed Google Scholar
- D. Sabes
You can also search for this author in PubMed Google Scholar
- R. Salerno
You can also search for this author in PubMed Google Scholar
- Y. Sirois
You can also search for this author in PubMed Google Scholar
- C. Thiebaux
You can also search for this author in PubMed Google Scholar
- C. Veelken
You can also search for this author in PubMed Google Scholar
- A. Zabi
You can also search for this author in PubMed Google Scholar
- J.-L. Agram
You can also search for this author in PubMed Google Scholar
- J. Andrea
You can also search for this author in PubMed Google Scholar
- D. Bloch
You can also search for this author in PubMed Google Scholar
- D. Bodin
You can also search for this author in PubMed Google Scholar
- J.-M. Brom
You can also search for this author in PubMed Google Scholar
- M. Cardaci
You can also search for this author in PubMed Google Scholar
- E. C. Chabert
You can also search for this author in PubMed Google Scholar
- C. Collard
You can also search for this author in PubMed Google Scholar
- E. Conte
You can also search for this author in PubMed Google Scholar
- F. Drouhin
You can also search for this author in PubMed Google Scholar
- C. Ferro
You can also search for this author in PubMed Google Scholar
- J.-C. Fontaine
You can also search for this author in PubMed Google Scholar
- D. Gelé
You can also search for this author in PubMed Google Scholar
- U. Goerlach
You can also search for this author in PubMed Google Scholar
- P. Juillot
You can also search for this author in PubMed Google Scholar
- M. Karim
You can also search for this author in PubMed Google Scholar
- A.-C. Le Bihan
You can also search for this author in PubMed Google Scholar
- P. Van Hove
You can also search for this author in PubMed Google Scholar
- F. Fassi
You can also search for this author in PubMed Google Scholar
- D. Mercier
You can also search for this author in PubMed Google Scholar
- C. Baty
You can also search for this author in PubMed Google Scholar
- S. Beauceron
You can also search for this author in PubMed Google Scholar
- N. Beaupere
You can also search for this author in PubMed Google Scholar
- M. Bedjidian
You can also search for this author in PubMed Google Scholar
- O. Bondu
You can also search for this author in PubMed Google Scholar
- G. Boudoul
You can also search for this author in PubMed Google Scholar
- D. Boumediene
You can also search for this author in PubMed Google Scholar
- H. Brun
You can also search for this author in PubMed Google Scholar
- J. Chasserat
You can also search for this author in PubMed Google Scholar
- R. Chierici
You can also search for this author in PubMed Google Scholar
- D. Contardo
You can also search for this author in PubMed Google Scholar
- P. Depasse
You can also search for this author in PubMed Google Scholar
- H. El Mamouni
You can also search for this author in PubMed Google Scholar
- A. Falkiewicz
You can also search for this author in PubMed Google Scholar
- J. Fay
You can also search for this author in PubMed Google Scholar
- S. Gascon
You can also search for this author in PubMed Google Scholar
- M. Gouzevitch
You can also search for this author in PubMed Google Scholar
- B. Ille
You can also search for this author in PubMed Google Scholar
- T. Kurca
You can also search for this author in PubMed Google Scholar
- T. Le Grand
You can also search for this author in PubMed Google Scholar
- M. Lethuillier
You can also search for this author in PubMed Google Scholar
- L. Mirabito
You can also search for this author in PubMed Google Scholar
- S. Perries
You can also search for this author in PubMed Google Scholar
- V. Sordini
You can also search for this author in PubMed Google Scholar
- S. Tosi
You can also search for this author in PubMed Google Scholar
- Y. Tschudi
You can also search for this author in PubMed Google Scholar
- P. Verdier
You can also search for this author in PubMed Google Scholar
- S. Viret
You can also search for this author in PubMed Google Scholar
- D. Lomidze
You can also search for this author in PubMed Google Scholar
- G. Anagnostou
You can also search for this author in PubMed Google Scholar
- S. Beranek
You can also search for this author in PubMed Google Scholar
- M. Edelhoff
You can also search for this author in PubMed Google Scholar
- L. Feld
You can also search for this author in PubMed Google Scholar
- N. Heracleous
You can also search for this author in PubMed Google Scholar
- O. Hindrichs
You can also search for this author in PubMed Google Scholar
- R. Jussen
You can also search for this author in PubMed Google Scholar
- K. Klein
You can also search for this author in PubMed Google Scholar
- J. Merz
You can also search for this author in PubMed Google Scholar
- A. Ostapchuk
You can also search for this author in PubMed Google Scholar
- A. Perieanu
You can also search for this author in PubMed Google Scholar
- F. Raupach
You can also search for this author in PubMed Google Scholar
- J. Sammet
You can also search for this author in PubMed Google Scholar
- S. Schael
You can also search for this author in PubMed Google Scholar
- D. Sprenger
You can also search for this author in PubMed Google Scholar
- H. Weber
You can also search for this author in PubMed Google Scholar
- B. Wittmer
You can also search for this author in PubMed Google Scholar
- V. Zhukov
You can also search for this author in PubMed Google Scholar
- M. Ata
You can also search for this author in PubMed Google Scholar
- J. Caudron
You can also search for this author in PubMed Google Scholar
- E. Dietz-Laursonn
You can also search for this author in PubMed Google Scholar
- M. Erdmann
You can also search for this author in PubMed Google Scholar
- A. Güth
You can also search for this author in PubMed Google Scholar
- T. Hebbeker
You can also search for this author in PubMed Google Scholar
- C. Heidemann
You can also search for this author in PubMed Google Scholar
- K. Hoepfner
You can also search for this author in PubMed Google Scholar
- T. Klimkovich
You can also search for this author in PubMed Google Scholar
- D. Klingebiel
You can also search for this author in PubMed Google Scholar
- P. Kreuzer
You can also search for this author in PubMed Google Scholar
- D. Lanske
You can also search for this author in PubMed Google Scholar
- J. Lingemann
You can also search for this author in PubMed Google Scholar
- C. Magass
You can also search for this author in PubMed Google Scholar
- M. Merschmeyer
You can also search for this author in PubMed Google Scholar
- A. Meyer
You can also search for this author in PubMed Google Scholar
- M. Olschewski
You can also search for this author in PubMed Google Scholar
- P. Papacz
You can also search for this author in PubMed Google Scholar
- H. Pieta
You can also search for this author in PubMed Google Scholar
- H. Reithler
You can also search for this author in PubMed Google Scholar
- S. A. Schmitz
You can also search for this author in PubMed Google Scholar
- L. Sonnenschein
You can also search for this author in PubMed Google Scholar
- J. Steggemann
You can also search for this author in PubMed Google Scholar
- D. Teyssier
You can also search for this author in PubMed Google Scholar
- M. Weber
You can also search for this author in PubMed Google Scholar
- M. Bontenackels
You can also search for this author in PubMed Google Scholar
- V. Cherepanov
You can also search for this author in PubMed Google Scholar
- M. Davids
You can also search for this author in PubMed Google Scholar
- G. Flügge
You can also search for this author in PubMed Google Scholar
- H. Geenen
You can also search for this author in PubMed Google Scholar
- M. Geisler
You can also search for this author in PubMed Google Scholar
- W. Haj Ahmad
You can also search for this author in PubMed Google Scholar
- F. Hoehle
You can also search for this author in PubMed Google Scholar
- B. Kargoll
You can also search for this author in PubMed Google Scholar
- T. Kress
You can also search for this author in PubMed Google Scholar
- Y. Kuessel
You can also search for this author in PubMed Google Scholar
- A. Linn
You can also search for this author in PubMed Google Scholar
- A. Nowack
You can also search for this author in PubMed Google Scholar
- L. Perchalla
You can also search for this author in PubMed Google Scholar
- O. Pooth
You can also search for this author in PubMed Google Scholar
- J. Rennefeld
You can also search for this author in PubMed Google Scholar
- P. Sauerland
You can also search for this author in PubMed Google Scholar
- A. Stahl
You can also search for this author in PubMed Google Scholar
- M. H. Zoeller
You can also search for this author in PubMed Google Scholar
- M. Aldaya Martin
You can also search for this author in PubMed Google Scholar
- W. Behrenhoff
You can also search for this author in PubMed Google Scholar
- U. Behrens
You can also search for this author in PubMed Google Scholar
- M. Bergholz
You can also search for this author in PubMed Google Scholar
- A. Bethani
You can also search for this author in PubMed Google Scholar
- K. Borras
You can also search for this author in PubMed Google Scholar
- A. Burgmeier
You can also search for this author in PubMed Google Scholar
- A. Cakir
You can also search for this author in PubMed Google Scholar
- L. Calligaris
You can also search for this author in PubMed Google Scholar
- A. Campbell
You can also search for this author in PubMed Google Scholar
- E. Castro
You can also search for this author in PubMed Google Scholar
- D. Dammann
You can also search for this author in PubMed Google Scholar
- G. Eckerlin
You can also search for this author in PubMed Google Scholar
- D. Eckstein
You can also search for this author in PubMed Google Scholar
- A. Flossdorf
You can also search for this author in PubMed Google Scholar
- G. Flucke
You can also search for this author in PubMed Google Scholar
- A. Geiser
You can also search for this author in PubMed Google Scholar
- J. Hauk
You can also search for this author in PubMed Google Scholar
- H. Jung
You can also search for this author in PubMed Google Scholar
- M. Kasemann
You can also search for this author in PubMed Google Scholar
- P. Katsas
You can also search for this author in PubMed Google Scholar
- C. Kleinwort
You can also search for this author in PubMed Google Scholar
- H. Kluge
You can also search for this author in PubMed Google Scholar
- A. Knutsson
You can also search for this author in PubMed Google Scholar
- M. Krämer
You can also search for this author in PubMed Google Scholar
- D. Krücker
You can also search for this author in PubMed Google Scholar
- E. Kuznetsova
You can also search for this author in PubMed Google Scholar
- W. Lange
You can also search for this author in PubMed Google Scholar
- W. Lohmann
You can also search for this author in PubMed Google Scholar
- B. Lutz
You can also search for this author in PubMed Google Scholar
- R. Mankel
You can also search for this author in PubMed Google Scholar
- I. Marfin
You can also search for this author in PubMed Google Scholar
- M. Marienfeld
You can also search for this author in PubMed Google Scholar
- I.-A. Melzer-Pellmann
You can also search for this author in PubMed Google Scholar
- A. B. Meyer
You can also search for this author in PubMed Google Scholar
- J. Mnich
You can also search for this author in PubMed Google Scholar
- A. Mussgiller
You can also search for this author in PubMed Google Scholar
- S. Naumann-Emme
You can also search for this author in PubMed Google Scholar
- J. Olzem
You can also search for this author in PubMed Google Scholar
- A. Petrukhin
You can also search for this author in PubMed Google Scholar
- D. Pitzl
You can also search for this author in PubMed Google Scholar
- A. Raspereza
You can also search for this author in PubMed Google Scholar
- P. M. Ribeiro Cipriano
You can also search for this author in PubMed Google Scholar
- M. Rosin
You can also search for this author in PubMed Google Scholar
- J. Salfeld-Nebgen
You can also search for this author in PubMed Google Scholar
- R. Schmidt
You can also search for this author in PubMed Google Scholar
- T. Schoerner-Sadenius
You can also search for this author in PubMed Google Scholar
- N. Sen
You can also search for this author in PubMed Google Scholar
- A. Spiridonov
You can also search for this author in PubMed Google Scholar
- M. Stein
You can also search for this author in PubMed Google Scholar
- J. Tomaszewska
You can also search for this author in PubMed Google Scholar
- R. Walsh
You can also search for this author in PubMed Google Scholar
- C. Wissing
You can also search for this author in PubMed Google Scholar
- C. Autermann
You can also search for this author in PubMed Google Scholar
- V. Blobel
You can also search for this author in PubMed Google Scholar
- S. Bobrovskyi
You can also search for this author in PubMed Google Scholar
- J. Draeger
You can also search for this author in PubMed Google Scholar
- H. Enderle
You can also search for this author in PubMed Google Scholar
- J. Erfle
You can also search for this author in PubMed Google Scholar
- U. Gebbert
You can also search for this author in PubMed Google Scholar
- M. Görner
You can also search for this author in PubMed Google Scholar
- T. Hermanns
You can also search for this author in PubMed Google Scholar
- R. S. Höing
You can also search for this author in PubMed Google Scholar
- K. Kaschube
You can also search for this author in PubMed Google Scholar
- G. Kaussen
You can also search for this author in PubMed Google Scholar
- H. Kirschenmann
You can also search for this author in PubMed Google Scholar
- R. Klanner
You can also search for this author in PubMed Google Scholar
- J. Lange
You can also search for this author in PubMed Google Scholar
- B. Mura
You can also search for this author in PubMed Google Scholar
- F. Nowak
You can also search for this author in PubMed Google Scholar
- N. Pietsch
You can also search for this author in PubMed Google Scholar
- C. Sander
You can also search for this author in PubMed Google Scholar
- H. Schettler
You can also search for this author in PubMed Google Scholar
- P. Schleper
You can also search for this author in PubMed Google Scholar
- E. Schlieckau
You can also search for this author in PubMed Google Scholar
- A. Schmidt
You can also search for this author in PubMed Google Scholar
- M. Schröder
You can also search for this author in PubMed Google Scholar
- T. Schum
You can also search for this author in PubMed Google Scholar
- H. Stadie
You can also search for this author in PubMed Google Scholar
- G. Steinbrück
You can also search for this author in PubMed Google Scholar
- J. Thomsen
You can also search for this author in PubMed Google Scholar
- C. Barth
You can also search for this author in PubMed Google Scholar
- J. Berger
You can also search for this author in PubMed Google Scholar
- T. Chwalek
You can also search for this author in PubMed Google Scholar
- W. De Boer
You can also search for this author in PubMed Google Scholar
- A. Dierlamm
You can also search for this author in PubMed Google Scholar
- G. Dirkes
You can also search for this author in PubMed Google Scholar
- M. Feindt
You can also search for this author in PubMed Google Scholar
- J. Gruschke
You can also search for this author in PubMed Google Scholar
- M. Guthoff
You can also search for this author in PubMed Google Scholar
- C. Hackstein
You can also search for this author in PubMed Google Scholar
- F. Hartmann
You can also search for this author in PubMed Google Scholar
- M. Heinrich
You can also search for this author in PubMed Google Scholar
- H. Held
You can also search for this author in PubMed Google Scholar
- K. H. Hoffmann
You can also search for this author in PubMed Google Scholar
- S. Honc
You can also search for this author in PubMed Google Scholar
- I. Katkov
You can also search for this author in PubMed Google Scholar
- J. R. Komaragiri
You can also search for this author in PubMed Google Scholar
- T. Kuhr
You can also search for this author in PubMed Google Scholar
- D. Martschei
You can also search for this author in PubMed Google Scholar
- S. Mueller
You can also search for this author in PubMed Google Scholar
- Th. Müller
You can also search for this author in PubMed Google Scholar
- M. Niegel
You can also search for this author in PubMed Google Scholar
- A. Nürnberg
You can also search for this author in PubMed Google Scholar
- O. Oberst
You can also search for this author in PubMed Google Scholar
- A. Oehler
You can also search for this author in PubMed Google Scholar
- J. Ott
You can also search for this author in PubMed Google Scholar
- T. Peiffer
You can also search for this author in PubMed Google Scholar
- G. Quast
You can also search for this author in PubMed Google Scholar
- K. Rabbertz
You can also search for this author in PubMed Google Scholar
- F. Ratnikov
You can also search for this author in PubMed Google Scholar
- N. Ratnikova
You can also search for this author in PubMed Google Scholar
- M. Renz
You can also search for this author in PubMed Google Scholar
- S. Röcker
You can also search for this author in PubMed Google Scholar
- C. Saout
You can also search for this author in PubMed Google Scholar
- A. Scheurer
You can also search for this author in PubMed Google Scholar
- P. Schieferdecker
You can also search for this author in PubMed Google Scholar
- F.-P. Schilling
You can also search for this author in PubMed Google Scholar
- M. Schmanau
You can also search for this author in PubMed Google Scholar
- G. Schott
You can also search for this author in PubMed Google Scholar
- H. J. Simonis
You can also search for this author in PubMed Google Scholar
- F. M. Stober
You can also search for this author in PubMed Google Scholar
- D. Troendle
You can also search for this author in PubMed Google Scholar
- J. Wagner-Kuhr
You can also search for this author in PubMed Google Scholar
- T. Weiler
You can also search for this author in PubMed Google Scholar
- M. Zeise
You can also search for this author in PubMed Google Scholar
- E. B. Ziebarth
You can also search for this author in PubMed Google Scholar
- G. Daskalakis
You can also search for this author in PubMed Google Scholar
- T. Geralis
You can also search for this author in PubMed Google Scholar
- S. Kesisoglou
You can also search for this author in PubMed Google Scholar
- A. Kyriakis
You can also search for this author in PubMed Google Scholar
- D. Loukas
You can also search for this author in PubMed Google Scholar
- I. Manolakos
You can also search for this author in PubMed Google Scholar
- A. Markou
You can also search for this author in PubMed Google Scholar
- C. Markou
You can also search for this author in PubMed Google Scholar
- C. Mavrommatis
You can also search for this author in PubMed Google Scholar
- E. Ntomari
You can also search for this author in PubMed Google Scholar
- L. Gouskos
You can also search for this author in PubMed Google Scholar
- T. J. Mertzimekis
You can also search for this author in PubMed Google Scholar
- A. Panagiotou
You can also search for this author in PubMed Google Scholar
- N. Saoulidou
You can also search for this author in PubMed Google Scholar
- E. Stiliaris
You can also search for this author in PubMed Google Scholar
- I. Evangelou
You can also search for this author in PubMed Google Scholar
- C. Foudas
You can also search for this author in PubMed Google Scholar
- P. Kokkas
You can also search for this author in PubMed Google Scholar
- N. Manthos
You can also search for this author in PubMed Google Scholar
- I. Papadopoulos
You can also search for this author in PubMed Google Scholar
- V. Patras
You can also search for this author in PubMed Google Scholar
- F. A. Triantis
You can also search for this author in PubMed Google Scholar
- A. Aranyi
You can also search for this author in PubMed Google Scholar
- G. Bencze
You can also search for this author in PubMed Google Scholar
- L. Boldizsar
You can also search for this author in PubMed Google Scholar
- C. Hajdu
You can also search for this author in PubMed Google Scholar
- P. Hidas
You can also search for this author in PubMed Google Scholar
- D. Horvath
You can also search for this author in PubMed Google Scholar
- A. Kapusi
You can also search for this author in PubMed Google Scholar
- K. Krajczar
You can also search for this author in PubMed Google Scholar
- F. Sikler
You can also search for this author in PubMed Google Scholar
- V. Veszpremi
You can also search for this author in PubMed Google Scholar
- G. Vesztergombi
You can also search for this author in PubMed Google Scholar
- N. Beni
You can also search for this author in PubMed Google Scholar
- J. Molnar
You can also search for this author in PubMed Google Scholar
- J. Palinkas
You can also search for this author in PubMed Google Scholar
- Z. Szillasi
You can also search for this author in PubMed Google Scholar
- J. Karancsi
You can also search for this author in PubMed Google Scholar
- P. Raics
You can also search for this author in PubMed Google Scholar
- Z. L. Trocsanyi
You can also search for this author in PubMed Google Scholar
- B. Ujvari
You can also search for this author in PubMed Google Scholar
- S. B. Beri
You can also search for this author in PubMed Google Scholar
- V. Bhatnagar
You can also search for this author in PubMed Google Scholar
- N. Dhingra
You can also search for this author in PubMed Google Scholar
- R. Gupta
You can also search for this author in PubMed Google Scholar
- M. Jindal
You can also search for this author in PubMed Google Scholar
- M. Kaur
You can also search for this author in PubMed Google Scholar
- J. M. Kohli
You can also search for this author in PubMed Google Scholar
- M. Z. Mehta
You can also search for this author in PubMed Google Scholar
- N. Nishu
You can also search for this author in PubMed Google Scholar
- L. K. Saini
You can also search for this author in PubMed Google Scholar
- A. Sharma
You can also search for this author in PubMed Google Scholar
- A. P. Singh
You can also search for this author in PubMed Google Scholar
- J. Singh
You can also search for this author in PubMed Google Scholar
- S. P. Singh
You can also search for this author in PubMed Google Scholar
- S. Ahuja
You can also search for this author in PubMed Google Scholar
- B. C. Choudhary
You can also search for this author in PubMed Google Scholar
- A. Kumar
You can also search for this author in PubMed Google Scholar
- A. Kumar
You can also search for this author in PubMed Google Scholar
- S. Malhotra
You can also search for this author in PubMed Google Scholar
- M. Naimuddin
You can also search for this author in PubMed Google Scholar
- K. Ranjan
You can also search for this author in PubMed Google Scholar
- V. Sharma
You can also search for this author in PubMed Google Scholar
- R. K. Shivpuri
You can also search for this author in PubMed Google Scholar
- S. Banerjee
You can also search for this author in PubMed Google Scholar
- S. Bhattacharya
You can also search for this author in PubMed Google Scholar
- S. Dutta
You can also search for this author in PubMed Google Scholar
- B. Gomber
You can also search for this author in PubMed Google Scholar
- Sa. Jain
You can also search for this author in PubMed Google Scholar
- Sh. Jain
You can also search for this author in PubMed Google Scholar
- R. Khurana
You can also search for this author in PubMed Google Scholar
- S. Sarkar
You can also search for this author in PubMed Google Scholar
- R. K. Choudhury
You can also search for this author in PubMed Google Scholar
- D. Dutta
You can also search for this author in PubMed Google Scholar
- S. Kailas
You can also search for this author in PubMed Google Scholar
- V. Kumar
You can also search for this author in PubMed Google Scholar
- A. K. Mohanty
You can also search for this author in PubMed Google Scholar
- L. M. Pant
You can also search for this author in PubMed Google Scholar
- P. Shukla
You can also search for this author in PubMed Google Scholar
- T. Aziz
You can also search for this author in PubMed Google Scholar
- S. Ganguly
You can also search for this author in PubMed Google Scholar
- M. Guchait
You can also search for this author in PubMed Google Scholar
- A. Gurtu
You can also search for this author in PubMed Google Scholar
- M. Maity
You can also search for this author in PubMed Google Scholar
- G. Majumder
You can also search for this author in PubMed Google Scholar
- K. Mazumdar
You can also search for this author in PubMed Google Scholar
- G. B. Mohanty
You can also search for this author in PubMed Google Scholar
- B. Parida
You can also search for this author in PubMed Google Scholar
- A. Saha
You can also search for this author in PubMed Google Scholar
- K. Sudhakar
You can also search for this author in PubMed Google Scholar
- N. Wickramage
You can also search for this author in PubMed Google Scholar
- S. Banerjee
You can also search for this author in PubMed Google Scholar
- S. Dugad
You can also search for this author in PubMed Google Scholar
- N. K. Mondal
You can also search for this author in PubMed Google Scholar
- H. Arfaei
You can also search for this author in PubMed Google Scholar
- H. Bakhshiansohi
You can also search for this author in PubMed Google Scholar
- S. M. Etesami
You can also search for this author in PubMed Google Scholar
- A. Fahim
You can also search for this author in PubMed Google Scholar
- M. Hashemi
You can also search for this author in PubMed Google Scholar
- H. Hesari
You can also search for this author in PubMed Google Scholar
- A. Jafari
You can also search for this author in PubMed Google Scholar
- M. Khakzad
You can also search for this author in PubMed Google Scholar
- A. Mohammadi
You can also search for this author in PubMed Google Scholar
- M. Mohammadi Najafabadi
You can also search for this author in PubMed Google Scholar
- S. Paktinat Mehdiabadi
You can also search for this author in PubMed Google Scholar
- B. Safarzadeh
You can also search for this author in PubMed Google Scholar
- M. Zeinali
You can also search for this author in PubMed Google Scholar
- M. Abbrescia
You can also search for this author in PubMed Google Scholar
- L. Barbone
You can also search for this author in PubMed Google Scholar
- C. Calabria
You can also search for this author in PubMed Google Scholar
- S. S. Chhibra
You can also search for this author in PubMed Google Scholar
- A. Colaleo
You can also search for this author in PubMed Google Scholar
- D. Creanza
You can also search for this author in PubMed Google Scholar
- N. De Filippis
You can also search for this author in PubMed Google Scholar
- M. De Palma
You can also search for this author in PubMed Google Scholar
- L. Fiore
You can also search for this author in PubMed Google Scholar
- G. Iaselli
You can also search for this author in PubMed Google Scholar
- L. Lusito
You can also search for this author in PubMed Google Scholar
- G. Maggi
You can also search for this author in PubMed Google Scholar
- M. Maggi
You can also search for this author in PubMed Google Scholar
- N. Manna
You can also search for this author in PubMed Google Scholar
- B. Marangelli
You can also search for this author in PubMed Google Scholar
- S. My
You can also search for this author in PubMed Google Scholar
- S. Nuzzo
You can also search for this author in PubMed Google Scholar
- N. Pacifico
You can also search for this author in PubMed Google Scholar
- A. Pompili
You can also search for this author in PubMed Google Scholar
- G. Pugliese
You can also search for this author in PubMed Google Scholar
- F. Romano
You can also search for this author in PubMed Google Scholar
- G. Selvaggi
You can also search for this author in PubMed Google Scholar
- L. Silvestris
You can also search for this author in PubMed Google Scholar
- G. Singh
You can also search for this author in PubMed Google Scholar
- S. Tupputi
You can also search for this author in PubMed Google Scholar
- G. Zito
You can also search for this author in PubMed Google Scholar
- G. Abbiendi
You can also search for this author in PubMed Google Scholar
- A. C. Benvenuti
You can also search for this author in PubMed Google Scholar
- D. Bonacorsi
You can also search for this author in PubMed Google Scholar
- S. Braibant-Giacomelli
You can also search for this author in PubMed Google Scholar
- L. Brigliadori
You can also search for this author in PubMed Google Scholar
- P. Capiluppi
You can also search for this author in PubMed Google Scholar
- A. Castro
You can also search for this author in PubMed Google Scholar
- F. R. Cavallo
You can also search for this author in PubMed Google Scholar
- M. Cuffiani
You can also search for this author in PubMed Google Scholar
- G. M. Dallavalle
You can also search for this author in PubMed Google Scholar
- F. Fabbri
You can also search for this author in PubMed Google Scholar
- A. Fanfani
You can also search for this author in PubMed Google Scholar
- D. Fasanella
You can also search for this author in PubMed Google Scholar
- P. Giacomelli
You can also search for this author in PubMed Google Scholar
- C. Grandi
You can also search for this author in PubMed Google Scholar
- S. Marcellini
You can also search for this author in PubMed Google Scholar
- G. Masetti
You can also search for this author in PubMed Google Scholar
- M. Meneghelli
You can also search for this author in PubMed Google Scholar
- A. Montanari
You can also search for this author in PubMed Google Scholar
- F. L. Navarria
You can also search for this author in PubMed Google Scholar
- F. Odorici
You can also search for this author in PubMed Google Scholar
- A. Perrotta
You can also search for this author in PubMed Google Scholar
- F. Primavera
You can also search for this author in PubMed Google Scholar
- A. M. Rossi
You can also search for this author in PubMed Google Scholar
- T. Rovelli
You can also search for this author in PubMed Google Scholar
- G. Siroli
You can also search for this author in PubMed Google Scholar
- R. Travaglini
You can also search for this author in PubMed Google Scholar
- S. Albergo
You can also search for this author in PubMed Google Scholar
- G. Cappello
You can also search for this author in PubMed Google Scholar
- M. Chiorboli
You can also search for this author in PubMed Google Scholar
- S. Costa
You can also search for this author in PubMed Google Scholar
- R. Potenza
You can also search for this author in PubMed Google Scholar
- A. Tricomi
You can also search for this author in PubMed Google Scholar
- C. Tuve
You can also search for this author in PubMed Google Scholar
- G. Barbagli
You can also search for this author in PubMed Google Scholar
- V. Ciulli
You can also search for this author in PubMed Google Scholar
- C. Civinini
You can also search for this author in PubMed Google Scholar
- R. D’Alessandro
You can also search for this author in PubMed Google Scholar
- E. Focardi
You can also search for this author in PubMed Google Scholar
- S. Frosali
You can also search for this author in PubMed Google Scholar
- E. Gallo
You can also search for this author in PubMed Google Scholar
- S. Gonzi
You can also search for this author in PubMed Google Scholar
- M. Meschini
You can also search for this author in PubMed Google Scholar
- S. Paoletti
You can also search for this author in PubMed Google Scholar
- G. Sguazzoni
You can also search for this author in PubMed Google Scholar
- A. Tropiano
You can also search for this author in PubMed Google Scholar
- L. Benussi
You can also search for this author in PubMed Google Scholar
- S. Bianco
You can also search for this author in PubMed Google Scholar
- S. Colafranceschi
You can also search for this author in PubMed Google Scholar
- F. Fabbri
You can also search for this author in PubMed Google Scholar
- D. Piccolo
You can also search for this author in PubMed Google Scholar
- P. Fabbricatore
You can also search for this author in PubMed Google Scholar
- R. Musenich
You can also search for this author in PubMed Google Scholar
- A. Benaglia
You can also search for this author in PubMed Google Scholar
- F. De Guio
You can also search for this author in PubMed Google Scholar
- L. Di Matteo
You can also search for this author in PubMed Google Scholar
- S. Fiorendi
You can also search for this author in PubMed Google Scholar
- S. Gennai
You can also search for this author in PubMed Google Scholar
- A. Ghezzi
You can also search for this author in PubMed Google Scholar
- S. Malvezzi
You can also search for this author in PubMed Google Scholar
- R. A. Manzoni
You can also search for this author in PubMed Google Scholar
- A. Martelli
You can also search for this author in PubMed Google Scholar
- A. Massironi
You can also search for this author in PubMed Google Scholar
- D. Menasce
You can also search for this author in PubMed Google Scholar
- L. Moroni
You can also search for this author in PubMed Google Scholar
- M. Paganoni
You can also search for this author in PubMed Google Scholar
- D. Pedrini
You can also search for this author in PubMed Google Scholar
- S. Ragazzi
You can also search for this author in PubMed Google Scholar
- N. Redaelli
You can also search for this author in PubMed Google Scholar
- S. Sala
You can also search for this author in PubMed Google Scholar
- T. Tabarelli de Fatis
You can also search for this author in PubMed Google Scholar
- S. Buontempo
You can also search for this author in PubMed Google Scholar
- C. A. Carrillo Montoya
You can also search for this author in PubMed Google Scholar
- N. Cavallo
You can also search for this author in PubMed Google Scholar
- A. De Cosa
You can also search for this author in PubMed Google Scholar
- O. Dogangun
You can also search for this author in PubMed Google Scholar
- F. Fabozzi
You can also search for this author in PubMed Google Scholar
- A. O. M. Iorio
You can also search for this author in PubMed Google Scholar
- L. Lista
You can also search for this author in PubMed Google Scholar
- M. Merola
You can also search for this author in PubMed Google Scholar
- P. Paolucci
You can also search for this author in PubMed Google Scholar
- P. Azzi
You can also search for this author in PubMed Google Scholar
- N. Bacchetta
You can also search for this author in PubMed Google Scholar
- P. Bellan
You can also search for this author in PubMed Google Scholar
- D. Bisello
You can also search for this author in PubMed Google Scholar
- A. Branca
You can also search for this author in PubMed Google Scholar
- R. Carlin
You can also search for this author in PubMed Google Scholar
- P. Checchia
You can also search for this author in PubMed Google Scholar
- T. Dorigo
You can also search for this author in PubMed Google Scholar
- U. Dosselli
You can also search for this author in PubMed Google Scholar
- F. Gasparini
You can also search for this author in PubMed Google Scholar
- U. Gasparini
You can also search for this author in PubMed Google Scholar
- A. Gozzelino
You can also search for this author in PubMed Google Scholar
- K. Kanishchev
You can also search for this author in PubMed Google Scholar
- S. Lacaprara
You can also search for this author in PubMed Google Scholar
- I. Lazzizzera
You can also search for this author in PubMed Google Scholar
- M. Margoni
You can also search for this author in PubMed Google Scholar
- M. Mazzucato
You can also search for this author in PubMed Google Scholar
- A. T. Meneguzzo
You can also search for this author in PubMed Google Scholar
- M. Nespolo
You can also search for this author in PubMed Google Scholar
- L. Perrozzi
You can also search for this author in PubMed Google Scholar
- N. Pozzobon
You can also search for this author in PubMed Google Scholar
- P. Ronchese
You can also search for this author in PubMed Google Scholar
- F. Simonetto
You can also search for this author in PubMed Google Scholar
- E. Torassa
You can also search for this author in PubMed Google Scholar
- M. Tosi
You can also search for this author in PubMed Google Scholar
- A. Triossi
You can also search for this author in PubMed Google Scholar
- S. Vanini
You can also search for this author in PubMed Google Scholar
- P. Zotto
You can also search for this author in PubMed Google Scholar
- G. Zumerle
You can also search for this author in PubMed Google Scholar
- U. Berzano
You can also search for this author in PubMed Google Scholar
- M. Gabusi
You can also search for this author in PubMed Google Scholar
- S. P. Ratti
You can also search for this author in PubMed Google Scholar
- C. Riccardi
You can also search for this author in PubMed Google Scholar
- P. Torre
You can also search for this author in PubMed Google Scholar
- P. Vitulo
You can also search for this author in PubMed Google Scholar
- M. Biasini
You can also search for this author in PubMed Google Scholar
- G. M. Bilei
You can also search for this author in PubMed Google Scholar
- B. Caponeri
You can also search for this author in PubMed Google Scholar
- L. Fanò
You can also search for this author in PubMed Google Scholar
- P. Lariccia
You can also search for this author in PubMed Google Scholar
- A. Lucaroni
You can also search for this author in PubMed Google Scholar
- G. Mantovani
You can also search for this author in PubMed Google Scholar
- M. Menichelli
You can also search for this author in PubMed Google Scholar
- A. Nappi
You can also search for this author in PubMed Google Scholar
- F. Romeo
You can also search for this author in PubMed Google Scholar
- A. Santocchia
You can also search for this author in PubMed Google Scholar
- S. Taroni
You can also search for this author in PubMed Google Scholar
- M. Valdata
You can also search for this author in PubMed Google Scholar
- P. Azzurri
You can also search for this author in PubMed Google Scholar
- G. Bagliesi
You can also search for this author in PubMed Google Scholar
- T. Boccali
You can also search for this author in PubMed Google Scholar
- G. Broccolo
You can also search for this author in PubMed Google Scholar
- R. Castaldi
You can also search for this author in PubMed Google Scholar
- R. T. D’Agnolo
You can also search for this author in PubMed Google Scholar
- R. Dell’Orso
You can also search for this author in PubMed Google Scholar
- F. Fiori
You can also search for this author in PubMed Google Scholar
- L. Foà
You can also search for this author in PubMed Google Scholar
- A. Giassi
You can also search for this author in PubMed Google Scholar
- A. Kraan
You can also search for this author in PubMed Google Scholar
- F. Ligabue
You can also search for this author in PubMed Google Scholar
- T. Lomtadze
You can also search for this author in PubMed Google Scholar
- L. Martini
You can also search for this author in PubMed Google Scholar
- A. Messineo
You can also search for this author in PubMed Google Scholar
- F. Palla
You can also search for this author in PubMed Google Scholar
- F. Palmonari
You can also search for this author in PubMed Google Scholar
- A. Rizzi
You can also search for this author in PubMed Google Scholar
- A. T. Serban
You can also search for this author in PubMed Google Scholar
- P. Spagnolo
You can also search for this author in PubMed Google Scholar
- R. Tenchini
You can also search for this author in PubMed Google Scholar
- G. Tonelli
You can also search for this author in PubMed Google Scholar
- A. Venturi
You can also search for this author in PubMed Google Scholar
- P. G. Verdini
You can also search for this author in PubMed Google Scholar
- L. Barone
You can also search for this author in PubMed Google Scholar
- F. Cavallari
You can also search for this author in PubMed Google Scholar
- D. Del Re
You can also search for this author in PubMed Google Scholar
- M. Diemoz
You can also search for this author in PubMed Google Scholar
- C. Fanelli
You can also search for this author in PubMed Google Scholar
- M. Grassi
You can also search for this author in PubMed Google Scholar
- E. Longo
You can also search for this author in PubMed Google Scholar
- P. Meridiani
You can also search for this author in PubMed Google Scholar
- F. Micheli
You can also search for this author in PubMed Google Scholar
- S. Nourbakhsh
You can also search for this author in PubMed Google Scholar
- G. Organtini
You can also search for this author in PubMed Google Scholar
- F. Pandolfi
You can also search for this author in PubMed Google Scholar
- R. Paramatti
You can also search for this author in PubMed Google Scholar
- S. Rahatlou
You can also search for this author in PubMed Google Scholar
- M. Sigamani
You can also search for this author in PubMed Google Scholar
- L. Soffi
You can also search for this author in PubMed Google Scholar
- N. Amapane
You can also search for this author in PubMed Google Scholar
- R. Arcidiacono
You can also search for this author in PubMed Google Scholar
- S. Argiro
You can also search for this author in PubMed Google Scholar
- M. Arneodo
You can also search for this author in PubMed Google Scholar
- C. Biino
You can also search for this author in PubMed Google Scholar
- C. Botta
You can also search for this author in PubMed Google Scholar
- N. Cartiglia
You can also search for this author in PubMed Google Scholar
- R. Castello
You can also search for this author in PubMed Google Scholar
- M. Costa
You can also search for this author in PubMed Google Scholar
- N. Demaria
You can also search for this author in PubMed Google Scholar
- A. Graziano
You can also search for this author in PubMed Google Scholar
- C. Mariotti
You can also search for this author in PubMed Google Scholar
- S. Maselli
You can also search for this author in PubMed Google Scholar
- E. Migliore
You can also search for this author in PubMed Google Scholar
- V. Monaco
You can also search for this author in PubMed Google Scholar
- M. Musich
You can also search for this author in PubMed Google Scholar
- M. M. Obertino
You can also search for this author in PubMed Google Scholar
- N. Pastrone
You can also search for this author in PubMed Google Scholar
- M. Pelliccioni
You can also search for this author in PubMed Google Scholar
- A. Potenza
You can also search for this author in PubMed Google Scholar
- A. Romero
You can also search for this author in PubMed Google Scholar
- M. Ruspa
You can also search for this author in PubMed Google Scholar
- R. Sacchi
You can also search for this author in PubMed Google Scholar
- V. Sola
You can also search for this author in PubMed Google Scholar
- A. Solano
You can also search for this author in PubMed Google Scholar
- A. Staiano
You can also search for this author in PubMed Google Scholar
- A. Vilela Pereira
You can also search for this author in PubMed Google Scholar
- S. Belforte
You can also search for this author in PubMed Google Scholar
- F. Cossutti
You can also search for this author in PubMed Google Scholar
- G. Della Ricca
You can also search for this author in PubMed Google Scholar
- B. Gobbo
You can also search for this author in PubMed Google Scholar
- M. Marone
You can also search for this author in PubMed Google Scholar
- D. Montanino
You can also search for this author in PubMed Google Scholar
- A. Penzo
You can also search for this author in PubMed Google Scholar
- S. G. Heo
You can also search for this author in PubMed Google Scholar
- S. K. Nam
You can also search for this author in PubMed Google Scholar
- S. Chang
You can also search for this author in PubMed Google Scholar
- J. Chung
You can also search for this author in PubMed Google Scholar
- D. H. Kim
You can also search for this author in PubMed Google Scholar
- G. N. Kim
You can also search for this author in PubMed Google Scholar
- J. E. Kim
You can also search for this author in PubMed Google Scholar
- D. J. Kong
You can also search for this author in PubMed Google Scholar
- H. Park
You can also search for this author in PubMed Google Scholar
- S. R. Ro
You can also search for this author in PubMed Google Scholar
- D. C. Son
You can also search for this author in PubMed Google Scholar
- J. Y. Kim
You can also search for this author in PubMed Google Scholar
- Zero J. Kim
You can also search for this author in PubMed Google Scholar
- S. Song
You can also search for this author in PubMed Google Scholar
- H. Y. Jo
You can also search for this author in PubMed Google Scholar
- S. Choi
You can also search for this author in PubMed Google Scholar
- D. Gyun
You can also search for this author in PubMed Google Scholar
- B. Hong
You can also search for this author in PubMed Google Scholar
- M. Jo
You can also search for this author in PubMed Google Scholar
- H. Kim
You can also search for this author in PubMed Google Scholar
- T. J. Kim
You can also search for this author in PubMed Google Scholar
- K. S. Lee
You can also search for this author in PubMed Google Scholar
- D. H. Moon
You can also search for this author in PubMed Google Scholar
- S. K. Park
You can also search for this author in PubMed Google Scholar
- E. Seo
You can also search for this author in PubMed Google Scholar
- K. S. Sim
You can also search for this author in PubMed Google Scholar
- M. Choi
You can also search for this author in PubMed Google Scholar
- S. Kang
You can also search for this author in PubMed Google Scholar
- H. Kim
You can also search for this author in PubMed Google Scholar
- J. H. Kim
You can also search for this author in PubMed Google Scholar
- C. Park
You can also search for this author in PubMed Google Scholar
- I. C. Park
You can also search for this author in PubMed Google Scholar
- S. Park
You can also search for this author in PubMed Google Scholar
- G. Ryu
You can also search for this author in PubMed Google Scholar
- Y. Cho
You can also search for this author in PubMed Google Scholar
- Y. Choi
You can also search for this author in PubMed Google Scholar
- Y. K. Choi
You can also search for this author in PubMed Google Scholar
- J. Goh
You can also search for this author in PubMed Google Scholar
- M. S. Kim
You can also search for this author in PubMed Google Scholar
- B. Lee
You can also search for this author in PubMed Google Scholar
- J. Lee
You can also search for this author in PubMed Google Scholar
- S. Lee
You can also search for this author in PubMed Google Scholar
- H. Seo
You can also search for this author in PubMed Google Scholar
- I. Yu
You can also search for this author in PubMed Google Scholar
- M. J. Bilinskas
You can also search for this author in PubMed Google Scholar
- I. Grigelionis
You can also search for this author in PubMed Google Scholar
- M. Janulis
You can also search for this author in PubMed Google Scholar
- H. Castilla-Valdez
You can also search for this author in PubMed Google Scholar
- E. De La Cruz-Burelo
You can also search for this author in PubMed Google Scholar
- I. Heredia-de La Cruz
You can also search for this author in PubMed Google Scholar
- R. Lopez-Fernandez
You can also search for this author in PubMed Google Scholar
- R. Magaña Villalba
You can also search for this author in PubMed Google Scholar
- J. Martínez-Ortega
You can also search for this author in PubMed Google Scholar
- A. Sánchez-Hernández
You can also search for this author in PubMed Google Scholar
- L. M. Villasenor-Cendejas
You can also search for this author in PubMed Google Scholar
- S. Carrillo Moreno
You can also search for this author in PubMed Google Scholar
- F. Vazquez Valencia
You can also search for this author in PubMed Google Scholar
- H. A. Salazar Ibarguen
You can also search for this author in PubMed Google Scholar
- E. Casimiro Linares
You can also search for this author in PubMed Google Scholar
- A. Morelos Pineda
You can also search for this author in PubMed Google Scholar
- M. A. Reyes-Santos
You can also search for this author in PubMed Google Scholar
- D. Krofcheck
You can also search for this author in PubMed Google Scholar
- A. J. Bell
You can also search for this author in PubMed Google Scholar
- P. H. Butler
You can also search for this author in PubMed Google Scholar
- R. Doesburg
You can also search for this author in PubMed Google Scholar
- S. Reucroft
You can also search for this author in PubMed Google Scholar
- H. Silverwood
You can also search for this author in PubMed Google Scholar
- M. Ahmad
You can also search for this author in PubMed Google Scholar
- M. I. Asghar
You can also search for this author in PubMed Google Scholar
- H. R. Hoorani
You can also search for this author in PubMed Google Scholar
- S. Khalid
You can also search for this author in PubMed Google Scholar
- W. A. Khan
You can also search for this author in PubMed Google Scholar
- T. Khurshid
You can also search for this author in PubMed Google Scholar
- S. Qazi
You can also search for this author in PubMed Google Scholar
- M. A. Shah
You can also search for this author in PubMed Google Scholar
- M. Shoaib
You can also search for this author in PubMed Google Scholar
- G. Brona
You can also search for this author in PubMed Google Scholar
- M. Cwiok
You can also search for this author in PubMed Google Scholar
- W. Dominik
You can also search for this author in PubMed Google Scholar
- K. Doroba
You can also search for this author in PubMed Google Scholar
- A. Kalinowski
You can also search for this author in PubMed Google Scholar
- M. Konecki
You can also search for this author in PubMed Google Scholar
- J. Krolikowski
You can also search for this author in PubMed Google Scholar
- H. Bialkowska
You can also search for this author in PubMed Google Scholar
- B. Boimska
You can also search for this author in PubMed Google Scholar
- T. Frueboes
You can also search for this author in PubMed Google Scholar
- R. Gokieli
You can also search for this author in PubMed Google Scholar
- M. Górski
You can also search for this author in PubMed Google Scholar
- M. Kazana
You can also search for this author in PubMed Google Scholar
- K. Nawrocki
You can also search for this author in PubMed Google Scholar
- K. Romanowska-Rybinska
You can also search for this author in PubMed Google Scholar
- M. Szleper
You can also search for this author in PubMed Google Scholar
- G. Wrochna
You can also search for this author in PubMed Google Scholar
- P. Zalewski
You can also search for this author in PubMed Google Scholar
- N. Almeida
You can also search for this author in PubMed Google Scholar
- P. Bargassa
You can also search for this author in PubMed Google Scholar
- A. David
You can also search for this author in PubMed Google Scholar
- P. Faccioli
You can also search for this author in PubMed Google Scholar
- P. G. Ferreira Parracho
You can also search for this author in PubMed Google Scholar
- M. Gallinaro
You can also search for this author in PubMed Google Scholar
- P. Musella
You can also search for this author in PubMed Google Scholar
- A. Nayak
You can also search for this author in PubMed Google Scholar
- J. Pela
You can also search for this author in PubMed Google Scholar
- P. Q. Ribeiro
You can also search for this author in PubMed Google Scholar
- J. Seixas
You can also search for this author in PubMed Google Scholar
- J. Varela
You can also search for this author in PubMed Google Scholar
- P. Vischia
You can also search for this author in PubMed Google Scholar
- S. Afanasiev
You can also search for this author in PubMed Google Scholar
- I. Belotelov
You can also search for this author in PubMed Google Scholar
- P. Bunin
You can also search for this author in PubMed Google Scholar
- M. Gavrilenko
You can also search for this author in PubMed Google Scholar
- I. Golutvin
You can also search for this author in PubMed Google Scholar
- I. Gorbunov
You can also search for this author in PubMed Google Scholar
- V. Karjavin
You can also search for this author in PubMed Google Scholar
- V. Konoplyanikov
You can also search for this author in PubMed Google Scholar
- G. Kozlov
You can also search for this author in PubMed Google Scholar
- A. Lanev
You can also search for this author in PubMed Google Scholar
- P. Moisenz
You can also search for this author in PubMed Google Scholar
- V. Palichik
You can also search for this author in PubMed Google Scholar
- V. Perelygin
You can also search for this author in PubMed Google Scholar
- S. Shmatov
You can also search for this author in PubMed Google Scholar
- V. Smirnov
You can also search for this author in PubMed Google Scholar
- A. Volodko
You can also search for this author in PubMed Google Scholar
- A. Zarubin
You can also search for this author in PubMed Google Scholar
- S. Evstyukhin
You can also search for this author in PubMed Google Scholar
- V. Golovtsov
You can also search for this author in PubMed Google Scholar
- Y. Ivanov
You can also search for this author in PubMed Google Scholar
- V. Kim
You can also search for this author in PubMed Google Scholar
- P. Levchenko
You can also search for this author in PubMed Google Scholar
- V. Murzin
You can also search for this author in PubMed Google Scholar
- V. Oreshkin
You can also search for this author in PubMed Google Scholar
- I. Smirnov
You can also search for this author in PubMed Google Scholar
- V. Sulimov
You can also search for this author in PubMed Google Scholar
- L. Uvarov
You can also search for this author in PubMed Google Scholar
- S. Vavilov
You can also search for this author in PubMed Google Scholar
- A. Vorobyev
You can also search for this author in PubMed Google Scholar
- An. Vorobyev
You can also search for this author in PubMed Google Scholar
- Yu. Andreev
You can also search for this author in PubMed Google Scholar
- A. Dermenev
You can also search for this author in PubMed Google Scholar
- S. Gninenko
You can also search for this author in PubMed Google Scholar
- N. Golubev
You can also search for this author in PubMed Google Scholar
- M. Kirsanov
You can also search for this author in PubMed Google Scholar
- N. Krasnikov
You can also search for this author in PubMed Google Scholar
- V. Matveev
You can also search for this author in PubMed Google Scholar
- G. Pivovarov
You can also search for this author in PubMed Google Scholar
- A. Toropin
You can also search for this author in PubMed Google Scholar
- S. Troitsky
You can also search for this author in PubMed Google Scholar
- V. Epshteyn
You can also search for this author in PubMed Google Scholar
- M. Erofeeva
You can also search for this author in PubMed Google Scholar
- V. Gavrilov
You can also search for this author in PubMed Google Scholar
- M. Kossov
You can also search for this author in PubMed Google Scholar
- A. Krokhotin
You can also search for this author in PubMed Google Scholar
- N. Lychkovskaya
You can also search for this author in PubMed Google Scholar
- V. Popov
You can also search for this author in PubMed Google Scholar
- G. Safronov
You can also search for this author in PubMed Google Scholar
- S. Semenov
You can also search for this author in PubMed Google Scholar
- V. Stolin
You can also search for this author in PubMed Google Scholar
- E. Vlasov
You can also search for this author in PubMed Google Scholar
- A. Zhokin
You can also search for this author in PubMed Google Scholar
- A. Belyaev
You can also search for this author in PubMed Google Scholar
- E. Boos
You can also search for this author in PubMed Google Scholar
- M. Dubinin
You can also search for this author in PubMed Google Scholar
- L. Dudko
You can also search for this author in PubMed Google Scholar
- A. Ershov
You can also search for this author in PubMed Google Scholar
- L. Khein
You can also search for this author in PubMed Google Scholar
- O. Kodolova
You can also search for this author in PubMed Google Scholar
- I. Lokhtin
You can also search for this author in PubMed Google Scholar
- A. Markina
You can also search for this author in PubMed Google Scholar
- S. Obraztsov
You can also search for this author in PubMed Google Scholar
- M. Perfilov
You can also search for this author in PubMed Google Scholar
- A. Proskuryakov
You can also search for this author in PubMed Google Scholar
- L. Sarycheva
You can also search for this author in PubMed Google Scholar
- V. Savrin
You can also search for this author in PubMed Google Scholar
- A. Snigirev
You can also search for this author in PubMed Google Scholar
- V. Andreev
You can also search for this author in PubMed Google Scholar
- M. Azarkin
You can also search for this author in PubMed Google Scholar
- I. Dremin
You can also search for this author in PubMed Google Scholar
- M. Kirakosyan
You can also search for this author in PubMed Google Scholar
- A. Leonidov
You can also search for this author in PubMed Google Scholar
- G. Mesyats
You can also search for this author in PubMed Google Scholar
- S. V. Rusakov
You can also search for this author in PubMed Google Scholar
- A. Vinogradov
You can also search for this author in PubMed Google Scholar
- I. Azhgirey
You can also search for this author in PubMed Google Scholar
- I. Bayshev
You can also search for this author in PubMed Google Scholar
- S. Bitioukov
You can also search for this author in PubMed Google Scholar
- V. Grishin
You can also search for this author in PubMed Google Scholar
- V. Kachanov
You can also search for this author in PubMed Google Scholar
- D. Konstantinov
You can also search for this author in PubMed Google Scholar
- A. Korablev
You can also search for this author in PubMed Google Scholar
- V. Krychkine
You can also search for this author in PubMed Google Scholar
- V. Petrov
You can also search for this author in PubMed Google Scholar
- R. Ryutin
You can also search for this author in PubMed Google Scholar
- A. Sobol
You can also search for this author in PubMed Google Scholar
- L. Tourtchanovitch
You can also search for this author in PubMed Google Scholar
- S. Troshin
You can also search for this author in PubMed Google Scholar
- N. Tyurin
You can also search for this author in PubMed Google Scholar
- A. Uzunian
You can also search for this author in PubMed Google Scholar
- A. Volkov
You can also search for this author in PubMed Google Scholar
- P. Adzic
You can also search for this author in PubMed Google Scholar
- M. Djordjevic
You can also search for this author in PubMed Google Scholar
- M. Ekmedzic
You can also search for this author in PubMed Google Scholar
- D. Krpic
You can also search for this author in PubMed Google Scholar
- J. Milosevic
You can also search for this author in PubMed Google Scholar
- M. Aguilar-Benitez
You can also search for this author in PubMed Google Scholar
- J. Alcaraz Maestre
You can also search for this author in PubMed Google Scholar
- P. Arce
You can also search for this author in PubMed Google Scholar
- C. Battilana
You can also search for this author in PubMed Google Scholar
- E. Calvo
You can also search for this author in PubMed Google Scholar
- M. Cerrada
You can also search for this author in PubMed Google Scholar
- M. Chamizo Llatas
You can also search for this author in PubMed Google Scholar
- N. Colino
You can also search for this author in PubMed Google Scholar
- B. De La Cruz
You can also search for this author in PubMed Google Scholar
- A. Delgado Peris
You can also search for this author in PubMed Google Scholar
- C. Diez Pardos
You can also search for this author in PubMed Google Scholar
- D. Domínguez Vázquez
You can also search for this author in PubMed Google Scholar
- C. Fernandez Bedoya
You can also search for this author in PubMed Google Scholar
- J. P. Fernández Ramos
You can also search for this author in PubMed Google Scholar
- A. Ferrando
You can also search for this author in PubMed Google Scholar
- J. Flix
You can also search for this author in PubMed Google Scholar
- M. C. Fouz
You can also search for this author in PubMed Google Scholar
- P. Garcia-Abia
You can also search for this author in PubMed Google Scholar
- O. Gonzalez Lopez
You can also search for this author in PubMed Google Scholar
- S. Goy Lopez
You can also search for this author in PubMed Google Scholar
- J. M. Hernandez
You can also search for this author in PubMed Google Scholar
- M. I. Josa
You can also search for this author in PubMed Google Scholar
- G. Merino
You can also search for this author in PubMed Google Scholar
- J. Puerta Pelayo
You can also search for this author in PubMed Google Scholar
- I. Redondo
You can also search for this author in PubMed Google Scholar
- L. Romero
You can also search for this author in PubMed Google Scholar
- J. Santaolalla
You can also search for this author in PubMed Google Scholar
- M. S. Soares
You can also search for this author in PubMed Google Scholar
- C. Willmott
You can also search for this author in PubMed Google Scholar
- C. Albajar
You can also search for this author in PubMed Google Scholar
- G. Codispoti
You can also search for this author in PubMed Google Scholar
- J. F. de Trocóniz
You can also search for this author in PubMed Google Scholar
- J. Cuevas
You can also search for this author in PubMed Google Scholar
- J. Fernandez Menendez
You can also search for this author in PubMed Google Scholar
- S. Folgueras
You can also search for this author in PubMed Google Scholar
- I. Gonzalez Caballero
You can also search for this author in PubMed Google Scholar
- L. Lloret Iglesias
You can also search for this author in PubMed Google Scholar
- J. Piedra Gomez
You can also search for this author in PubMed Google Scholar
- J. M. Vizan Garcia
You can also search for this author in PubMed Google Scholar
- J. A. Brochero Cifuentes
You can also search for this author in PubMed Google Scholar
- I. J. Cabrillo
You can also search for this author in PubMed Google Scholar
- A. Calderon
You can also search for this author in PubMed Google Scholar
- S. H. Chuang
You can also search for this author in PubMed Google Scholar
- J. Duarte Campderros
You can also search for this author in PubMed Google Scholar
- M. Felcini
You can also search for this author in PubMed Google Scholar
- M. Fernandez
You can also search for this author in PubMed Google Scholar
- G. Gomez
You can also search for this author in PubMed Google Scholar
- J. Gonzalez Sanchez
You can also search for this author in PubMed Google Scholar
- C. Jorda
You can also search for this author in PubMed Google Scholar
- P. Lobelle Pardo
You can also search for this author in PubMed Google Scholar
- A. Lopez Virto
You can also search for this author in PubMed Google Scholar
- J. Marco
You can also search for this author in PubMed Google Scholar
- R. Marco
You can also search for this author in PubMed Google Scholar
- C. Martinez Rivero
You can also search for this author in PubMed Google Scholar
- F. Matorras
You can also search for this author in PubMed Google Scholar
- F. J. Munoz Sanchez
You can also search for this author in PubMed Google Scholar
- T. Rodrigo
You can also search for this author in PubMed Google Scholar
- A. Y. Rodríguez-Marrero
You can also search for this author in PubMed Google Scholar
- A. Ruiz-Jimeno
You can also search for this author in PubMed Google Scholar
- L. Scodellaro
You can also search for this author in PubMed Google Scholar
- M. Sobron Sanudo
You can also search for this author in PubMed Google Scholar
- I. Vila
You can also search for this author in PubMed Google Scholar
- R. Vilar Cortabitarte
You can also search for this author in PubMed Google Scholar
- D. Abbaneo
You can also search for this author in PubMed Google Scholar
- E. Auffray
You can also search for this author in PubMed Google Scholar
- G. Auzinger
You can also search for this author in PubMed Google Scholar
- P. Baillon
You can also search for this author in PubMed Google Scholar
- A. H. Ball
You can also search for this author in PubMed Google Scholar
- D. Barney
You can also search for this author in PubMed Google Scholar
- C. Bernet
You can also search for this author in PubMed Google Scholar
- W. Bialas
You can also search for this author in PubMed Google Scholar
- G. Bianchi
You can also search for this author in PubMed Google Scholar
- P. Bloch
You can also search for this author in PubMed Google Scholar
- A. Bocci
You can also search for this author in PubMed Google Scholar
- H. Breuker
You can also search for this author in PubMed Google Scholar
- K. Bunkowski
You can also search for this author in PubMed Google Scholar
- T. Camporesi
You can also search for this author in PubMed Google Scholar
- G. Cerminara
You can also search for this author in PubMed Google Scholar
- T. Christiansen
You can also search for this author in PubMed Google Scholar
- J. A. Coarasa Perez
You can also search for this author in PubMed Google Scholar
- B. Curé
You can also search for this author in PubMed Google Scholar
- D. D’Enterria
You can also search for this author in PubMed Google Scholar
- A. De Roeck
You can also search for this author in PubMed Google Scholar
- S. Di Guida
You can also search for this author in PubMed Google Scholar
- M. Dobson
You can also search for this author in PubMed Google Scholar
- N. Dupont-Sagorin
You can also search for this author in PubMed Google Scholar
- A. Elliott-Peisert
You can also search for this author in PubMed Google Scholar
- B. Frisch
You can also search for this author in PubMed Google Scholar
- W. Funk
You can also search for this author in PubMed Google Scholar
- A. Gaddi
You can also search for this author in PubMed Google Scholar
- G. Georgiou
You can also search for this author in PubMed Google Scholar
- H. Gerwig
You can also search for this author in PubMed Google Scholar
- M. Giffels
You can also search for this author in PubMed Google Scholar
- D. Gigi
You can also search for this author in PubMed Google Scholar
- K. Gill
You can also search for this author in PubMed Google Scholar
- D. Giordano
You can also search for this author in PubMed Google Scholar
- M. Giunta
You can also search for this author in PubMed Google Scholar
- F. Glege
You can also search for this author in PubMed Google Scholar
- R. Gomez-Reino Garrido
You can also search for this author in PubMed Google Scholar
- P. Govoni
You can also search for this author in PubMed Google Scholar
- S. Gowdy
You can also search for this author in PubMed Google Scholar
- R. Guida
You can also search for this author in PubMed Google Scholar
- L. Guiducci
You can also search for this author in PubMed Google Scholar
- M. Hansen
You can also search for this author in PubMed Google Scholar
- P. Harris
You can also search for this author in PubMed Google Scholar
- C. Hartl
You can also search for this author in PubMed Google Scholar
- J. Harvey
You can also search for this author in PubMed Google Scholar
- B. Hegner
You can also search for this author in PubMed Google Scholar
- A. Hinzmann
You can also search for this author in PubMed Google Scholar
- H. F. Hoffmann
You can also search for this author in PubMed Google Scholar
- V. Innocente
You can also search for this author in PubMed Google Scholar
- P. Janot
You can also search for this author in PubMed Google Scholar
- K. Kaadze
You can also search for this author in PubMed Google Scholar
- E. Karavakis
You can also search for this author in PubMed Google Scholar
- K. Kousouris
You can also search for this author in PubMed Google Scholar
- P. Lecoq
You can also search for this author in PubMed Google Scholar
- P. Lenzi
You can also search for this author in PubMed Google Scholar
- C. Lourenço
You can also search for this author in PubMed Google Scholar
- T. Mäki
You can also search for this author in PubMed Google Scholar
- M. Malberti
You can also search for this author in PubMed Google Scholar
- L. Malgeri
You can also search for this author in PubMed Google Scholar
- M. Mannelli
You can also search for this author in PubMed Google Scholar
- L. Masetti
You can also search for this author in PubMed Google Scholar
- G. Mavromanolakis
You can also search for this author in PubMed Google Scholar
- F. Meijers
You can also search for this author in PubMed Google Scholar
- S. Mersi
You can also search for this author in PubMed Google Scholar
- E. Meschi
You can also search for this author in PubMed Google Scholar
- R. Moser
You can also search for this author in PubMed Google Scholar
- M. U. Mozer
You can also search for this author in PubMed Google Scholar
- M. Mulders
You can also search for this author in PubMed Google Scholar
- E. Nesvold
You can also search for this author in PubMed Google Scholar
- M. Nguyen
You can also search for this author in PubMed Google Scholar
- T. Orimoto
You can also search for this author in PubMed Google Scholar
- L. Orsini
You can also search for this author in PubMed Google Scholar
- E. Palencia Cortezon
You can also search for this author in PubMed Google Scholar
- E. Perez
You can also search for this author in PubMed Google Scholar
- A. Petrilli
You can also search for this author in PubMed Google Scholar
- A. Pfeiffer
You can also search for this author in PubMed Google Scholar
- M. Pierini
You can also search for this author in PubMed Google Scholar
- M. Pimiä
You can also search for this author in PubMed Google Scholar
- D. Piparo
You can also search for this author in PubMed Google Scholar
- G. Polese
You can also search for this author in PubMed Google Scholar
- L. Quertenmont
You can also search for this author in PubMed Google Scholar
- A. Racz
You can also search for this author in PubMed Google Scholar
- W. Reece
You can also search for this author in PubMed Google Scholar
- J. Rodrigues Antunes
You can also search for this author in PubMed Google Scholar
- G. Rolandi
You can also search for this author in PubMed Google Scholar
- T. Rommerskirchen
You can also search for this author in PubMed Google Scholar
- C. Rovelli
You can also search for this author in PubMed Google Scholar
- M. Rovere
You can also search for this author in PubMed Google Scholar
- H. Sakulin
You can also search for this author in PubMed Google Scholar
- F. Santanastasio
You can also search for this author in PubMed Google Scholar
- C. Schäfer
You can also search for this author in PubMed Google Scholar
- C. Schwick
You can also search for this author in PubMed Google Scholar
- I. Segoni
You can also search for this author in PubMed Google Scholar
- A. Sharma
You can also search for this author in PubMed Google Scholar
- P. Siegrist
You can also search for this author in PubMed Google Scholar
- P. Silva
You can also search for this author in PubMed Google Scholar
- M. Simon
You can also search for this author in PubMed Google Scholar
- P. Sphicas
You can also search for this author in PubMed Google Scholar
- D. Spiga
You can also search for this author in PubMed Google Scholar
- M. Spiropulu
You can also search for this author in PubMed Google Scholar
- M. Stoye
You can also search for this author in PubMed Google Scholar
- A. Tsirou
You can also search for this author in PubMed Google Scholar
- G. I. Veres
You can also search for this author in PubMed Google Scholar
- P. Vichoudis
You can also search for this author in PubMed Google Scholar
- H. K. Wöhri
You can also search for this author in PubMed Google Scholar
- S. D. Worm
You can also search for this author in PubMed Google Scholar
- W. D. Zeuner
You can also search for this author in PubMed Google Scholar
- W. Bertl
You can also search for this author in PubMed Google Scholar
- K. Deiters
You can also search for this author in PubMed Google Scholar
- W. Erdmann
You can also search for this author in PubMed Google Scholar
- K. Gabathuler
You can also search for this author in PubMed Google Scholar
- R. Horisberger
You can also search for this author in PubMed Google Scholar
- Q. Ingram
You can also search for this author in PubMed Google Scholar
- H. C. Kaestli
You can also search for this author in PubMed Google Scholar
- S. König
You can also search for this author in PubMed Google Scholar
- D. Kotlinski
You can also search for this author in PubMed Google Scholar
- U. Langenegger
You can also search for this author in PubMed Google Scholar
- F. Meier
You can also search for this author in PubMed Google Scholar
- D. Renker
You can also search for this author in PubMed Google Scholar
- T. Rohe
You can also search for this author in PubMed Google Scholar
- J. Sibille
You can also search for this author in PubMed Google Scholar
- L. Bäni
You can also search for this author in PubMed Google Scholar
- P. Bortignon
You can also search for this author in PubMed Google Scholar
- M. A. Buchmann
You can also search for this author in PubMed Google Scholar
- B. Casal
You can also search for this author in PubMed Google Scholar
- N. Chanon
You can also search for this author in PubMed Google Scholar
- Z. Chen
You can also search for this author in PubMed Google Scholar
- A. Deisher
You can also search for this author in PubMed Google Scholar
- G. Dissertori
You can also search for this author in PubMed Google Scholar
- M. Dittmar
You can also search for this author in PubMed Google Scholar
- M. Dünser
You can also search for this author in PubMed Google Scholar
- J. Eugster
You can also search for this author in PubMed Google Scholar
- K. Freudenreich
You can also search for this author in PubMed Google Scholar
- C. Grab
You can also search for this author in PubMed Google Scholar
- P. Lecomte
You can also search for this author in PubMed Google Scholar
- W. Lustermann
You can also search for this author in PubMed Google Scholar
- P. Martinez Ruiz del Arbol
You can also search for this author in PubMed Google Scholar
- N. Mohr
You can also search for this author in PubMed Google Scholar
- F. Moortgat
You can also search for this author in PubMed Google Scholar
- C. Nägeli
You can also search for this author in PubMed Google Scholar
- P. Nef
You can also search for this author in PubMed Google Scholar
- F. Nessi-Tedaldi
You can also search for this author in PubMed Google Scholar
- L. Pape
You can also search for this author in PubMed Google Scholar
- F. Pauss
You can also search for this author in PubMed Google Scholar
- M. Peruzzi
You can also search for this author in PubMed Google Scholar
- F. J. Ronga
You can also search for this author in PubMed Google Scholar
- M. Rossini
You can also search for this author in PubMed Google Scholar
- L. Sala
You can also search for this author in PubMed Google Scholar
- A. K. Sanchez
You can also search for this author in PubMed Google Scholar
- M.-C. Sawley
You can also search for this author in PubMed Google Scholar
- A. Starodumov
You can also search for this author in PubMed Google Scholar
- B. Stieger
You can also search for this author in PubMed Google Scholar
- M. Takahashi
You can also search for this author in PubMed Google Scholar
- L. Tauscher
You can also search for this author in PubMed Google Scholar
- A. Thea
You can also search for this author in PubMed Google Scholar
- K. Theofilatos
You can also search for this author in PubMed Google Scholar
- D. Treille
You can also search for this author in PubMed Google Scholar
- C. Urscheler
You can also search for this author in PubMed Google Scholar
- R. Wallny
You can also search for this author in PubMed Google Scholar
- H. A. Weber
You can also search for this author in PubMed Google Scholar
- L. Wehrli
You can also search for this author in PubMed Google Scholar
- J. Weng
You can also search for this author in PubMed Google Scholar
- E. Aguilo
You can also search for this author in PubMed Google Scholar
- C. Amsler
You can also search for this author in PubMed Google Scholar
- V. Chiochia
You can also search for this author in PubMed Google Scholar
- S. De Visscher
You can also search for this author in PubMed Google Scholar
- C. Favaro
You can also search for this author in PubMed Google Scholar
- M. Ivova Rikova
You can also search for this author in PubMed Google Scholar
- B. Millan Mejias
You can also search for this author in PubMed Google Scholar
- P. Otiougova
You can also search for this author in PubMed Google Scholar
- P. Robmann
You can also search for this author in PubMed Google Scholar
- H. Snoek
You can also search for this author in PubMed Google Scholar
- M. Verzetti
You can also search for this author in PubMed Google Scholar
- Y. H. Chang
You can also search for this author in PubMed Google Scholar
- K. H. Chen
You can also search for this author in PubMed Google Scholar
- C. M. Kuo
You can also search for this author in PubMed Google Scholar
- S. W. Li
You can also search for this author in PubMed Google Scholar
- W. Lin
You can also search for this author in PubMed Google Scholar
- Z. K. Liu
You can also search for this author in PubMed Google Scholar
- Y. J. Lu
You can also search for this author in PubMed Google Scholar
- D. Mekterovic
You can also search for this author in PubMed Google Scholar
- R. Volpe
You can also search for this author in PubMed Google Scholar
- S. S. Yu
You can also search for this author in PubMed Google Scholar
- P. Bartalini
You can also search for this author in PubMed Google Scholar
- P. Chang
You can also search for this author in PubMed Google Scholar
- Y. H. Chang
You can also search for this author in PubMed Google Scholar
- Y. W. Chang
You can also search for this author in PubMed Google Scholar
- Y. Chao
You can also search for this author in PubMed Google Scholar
- K. F. Chen
You can also search for this author in PubMed Google Scholar
- C. Dietz
You can also search for this author in PubMed Google Scholar
- U. Grundler
You can also search for this author in PubMed Google Scholar
- W.-S. Hou
You can also search for this author in PubMed Google Scholar
- Y. Hsiung
You can also search for this author in PubMed Google Scholar
- K. Y. Kao
You can also search for this author in PubMed Google Scholar
- Y. J. Lei
You can also search for this author in PubMed Google Scholar
- R.-S. Lu
You can also search for this author in PubMed Google Scholar
- D. Majumder
You can also search for this author in PubMed Google Scholar
- E. Petrakou
You can also search for this author in PubMed Google Scholar
- X. Shi
You can also search for this author in PubMed Google Scholar
- J. G. Shiu
You can also search for this author in PubMed Google Scholar
- Y. M. Tzeng
You can also search for this author in PubMed Google Scholar
- M. Wang
You can also search for this author in PubMed Google Scholar
- A. Adiguzel
You can also search for this author in PubMed Google Scholar
- M. N. Bakirci
You can also search for this author in PubMed Google Scholar
- S. Cerci
You can also search for this author in PubMed Google Scholar
- C. Dozen
You can also search for this author in PubMed Google Scholar
- I. Dumanoglu
You can also search for this author in PubMed Google Scholar
- E. Eskut
You can also search for this author in PubMed Google Scholar
- S. Girgis
You can also search for this author in PubMed Google Scholar
- G. Gokbulut
You can also search for this author in PubMed Google Scholar
- I. Hos
You can also search for this author in PubMed Google Scholar
- E. E. Kangal
You can also search for this author in PubMed Google Scholar
- G. Karapinar
You can also search for this author in PubMed Google Scholar
- A. Kayis Topaksu
You can also search for this author in PubMed Google Scholar
- G. Onengut
You can also search for this author in PubMed Google Scholar
- K. Ozdemir
You can also search for this author in PubMed Google Scholar
- S. Ozturk
You can also search for this author in PubMed Google Scholar
- A. Polatoz
You can also search for this author in PubMed Google Scholar
- K. Sogut
You can also search for this author in PubMed Google Scholar
- D. Sunar Cerci
You can also search for this author in PubMed Google Scholar
- B. Tali
You can also search for this author in PubMed Google Scholar
- H. Topakli
You can also search for this author in PubMed Google Scholar
- D. Uzun
You can also search for this author in PubMed Google Scholar
- L. N. Vergili
You can also search for this author in PubMed Google Scholar
- M. Vergili
You can also search for this author in PubMed Google Scholar
- I. V. Akin
You can also search for this author in PubMed Google Scholar
- T. Aliev
You can also search for this author in PubMed Google Scholar
- B. Bilin
You can also search for this author in PubMed Google Scholar
- S. Bilmis
You can also search for this author in PubMed Google Scholar
- M. Deniz
You can also search for this author in PubMed Google Scholar
- H. Gamsizkan
You can also search for this author in PubMed Google Scholar
- A. M. Guler
You can also search for this author in PubMed Google Scholar
- K. Ocalan
You can also search for this author in PubMed Google Scholar
- A. Ozpineci
You can also search for this author in PubMed Google Scholar
- M. Serin
You can also search for this author in PubMed Google Scholar
- R. Sever
You can also search for this author in PubMed Google Scholar
- U. E. Surat
You can also search for this author in PubMed Google Scholar
- M. Yalvac
You can also search for this author in PubMed Google Scholar
- E. Yildirim
You can also search for this author in PubMed Google Scholar
- M. Zeyrek
You can also search for this author in PubMed Google Scholar
- M. Deliomeroglu
You can also search for this author in PubMed Google Scholar
- E. Gülmez
You can also search for this author in PubMed Google Scholar
- B. Isildak
You can also search for this author in PubMed Google Scholar
- M. Kaya
You can also search for this author in PubMed Google Scholar
- O. Kaya
You can also search for this author in PubMed Google Scholar
- S. Ozkorucuklu
You can also search for this author in PubMed Google Scholar
- N. Sonmez
You can also search for this author in PubMed Google Scholar
- L. Levchuk
You can also search for this author in PubMed Google Scholar
- F. Bostock
You can also search for this author in PubMed Google Scholar
- J. J. Brooke
You can also search for this author in PubMed Google Scholar
- E. Clement
You can also search for this author in PubMed Google Scholar
- D. Cussans
You can also search for this author in PubMed Google Scholar
- H. Flacher
You can also search for this author in PubMed Google Scholar
- R. Frazier
You can also search for this author in PubMed Google Scholar
- J. Goldstein
You can also search for this author in PubMed Google Scholar
- M. Grimes
You can also search for this author in PubMed Google Scholar
- G. P. Heath
You can also search for this author in PubMed Google Scholar
- H. F. Heath
You can also search for this author in PubMed Google Scholar
- L. Kreczko
You can also search for this author in PubMed Google Scholar
- S. Metson
You can also search for this author in PubMed Google Scholar
- D. M. Newbold
You can also search for this author in PubMed Google Scholar
- K. Nirunpong
You can also search for this author in PubMed Google Scholar
- A. Poll
You can also search for this author in PubMed Google Scholar
- S. Senkin
You can also search for this author in PubMed Google Scholar
- V. J. Smith
You can also search for this author in PubMed Google Scholar
- T. Williams
You can also search for this author in PubMed Google Scholar
- L. Basso
You can also search for this author in PubMed Google Scholar
- K. W. Bell
You can also search for this author in PubMed Google Scholar
- A. Belyaev
You can also search for this author in PubMed Google Scholar
- C. Brew
You can also search for this author in PubMed Google Scholar
- R. M. Brown
You can also search for this author in PubMed Google Scholar
- D. J. A. Cockerill
You can also search for this author in PubMed Google Scholar
- J. A. Coughlan
You can also search for this author in PubMed Google Scholar
- K. Harder
You can also search for this author in PubMed Google Scholar
- S. Harper
You can also search for this author in PubMed Google Scholar
- J. Jackson
You can also search for this author in PubMed Google Scholar
- B. W. Kennedy
You can also search for this author in PubMed Google Scholar
- E. Olaiya
You can also search for this author in PubMed Google Scholar
- D. Petyt
You can also search for this author in PubMed Google Scholar
- B. C. Radburn-Smith
You can also search for this author in PubMed Google Scholar
- C. H. Shepherd-Themistocleous
You can also search for this author in PubMed Google Scholar
- I. R. Tomalin
You can also search for this author in PubMed Google Scholar
- W. J. Womersley
You can also search for this author in PubMed Google Scholar
- R. Bainbridge
You can also search for this author in PubMed Google Scholar
- G. Ball
You can also search for this author in PubMed Google Scholar
- R. Beuselinck
You can also search for this author in PubMed Google Scholar
- O. Buchmuller
You can also search for this author in PubMed Google Scholar
- D. Colling
You can also search for this author in PubMed Google Scholar
- N. Cripps
You can also search for this author in PubMed Google Scholar
- M. Cutajar
You can also search for this author in PubMed Google Scholar
- P. Dauncey
You can also search for this author in PubMed Google Scholar
- G. Davies
You can also search for this author in PubMed Google Scholar
- M. Della Negra
You can also search for this author in PubMed Google Scholar
- W. Ferguson
You can also search for this author in PubMed Google Scholar
- J. Fulcher
You can also search for this author in PubMed Google Scholar
- D. Futyan
You can also search for this author in PubMed Google Scholar
- A. Gilbert
You can also search for this author in PubMed Google Scholar
- A. Guneratne Bryer
You can also search for this author in PubMed Google Scholar
- G. Hall
You can also search for this author in PubMed Google Scholar
- Z. Hatherell
You can also search for this author in PubMed Google Scholar
- J. Hays
You can also search for this author in PubMed Google Scholar
- G. Iles
You can also search for this author in PubMed Google Scholar
- M. Jarvis
You can also search for this author in PubMed Google Scholar
- G. Karapostoli
You can also search for this author in PubMed Google Scholar
- L. Lyons
You can also search for this author in PubMed Google Scholar
- A.-M. Magnan
You can also search for this author in PubMed Google Scholar
- J. Marrouche
You can also search for this author in PubMed Google Scholar
- B. Mathias
You can also search for this author in PubMed Google Scholar
- R. Nandi
You can also search for this author in PubMed Google Scholar
- J. Nash
You can also search for this author in PubMed Google Scholar
- A. Nikitenko
You can also search for this author in PubMed Google Scholar
- A. Papageorgiou
You can also search for this author in PubMed Google Scholar
- M. Pesaresi
You can also search for this author in PubMed Google Scholar
- K. Petridis
You can also search for this author in PubMed Google Scholar
- M. Pioppi
You can also search for this author in PubMed Google Scholar
- D. M. Raymond
You can also search for this author in PubMed Google Scholar
- S. Rogerson
You can also search for this author in PubMed Google Scholar
- N. Rompotis
You can also search for this author in PubMed Google Scholar
- A. Rose
You can also search for this author in PubMed Google Scholar
- M. J. Ryan
You can also search for this author in PubMed Google Scholar
- C. Seez
You can also search for this author in PubMed Google Scholar
- A. Sparrow
You can also search for this author in PubMed Google Scholar
- A. Tapper
You can also search for this author in PubMed Google Scholar
- S. Tourneur
You can also search for this author in PubMed Google Scholar
- M. Vazquez Acosta
You can also search for this author in PubMed Google Scholar
- T. Virdee
You can also search for this author in PubMed Google Scholar
- S. Wakefield
You can also search for this author in PubMed Google Scholar
- N. Wardle
You can also search for this author in PubMed Google Scholar
- D. Wardrope
You can also search for this author in PubMed Google Scholar
- T. Whyntie
You can also search for this author in PubMed Google Scholar
- M. Barrett
You can also search for this author in PubMed Google Scholar
- M. Chadwick
You can also search for this author in PubMed Google Scholar
- J. E. Cole
You can also search for this author in PubMed Google Scholar
- P. R. Hobson
You can also search for this author in PubMed Google Scholar
- A. Khan
You can also search for this author in PubMed Google Scholar
- P. Kyberd
You can also search for this author in PubMed Google Scholar
- D. Leslie
You can also search for this author in PubMed Google Scholar
- W. Martin
You can also search for this author in PubMed Google Scholar
- I. D. Reid
You can also search for this author in PubMed Google Scholar
- P. Symonds
You can also search for this author in PubMed Google Scholar
- L. Teodorescu
You can also search for this author in PubMed Google Scholar
- M. Turner
You can also search for this author in PubMed Google Scholar
- K. Hatakeyama
You can also search for this author in PubMed Google Scholar
- H. Liu
You can also search for this author in PubMed Google Scholar
- T. Scarborough
You can also search for this author in PubMed Google Scholar
- C. Henderson
You can also search for this author in PubMed Google Scholar
- A. Avetisyan
You can also search for this author in PubMed Google Scholar
- T. Bose
You can also search for this author in PubMed Google Scholar
- E. Carrera Jarrin
You can also search for this author in PubMed Google Scholar
- C. Fantasia
You can also search for this author in PubMed Google Scholar
- A. Heister
You can also search for this author in PubMed Google Scholar
- J. St. John
You can also search for this author in PubMed Google Scholar
- P. Lawson
You can also search for this author in PubMed Google Scholar
- D. Lazic
You can also search for this author in PubMed Google Scholar
- J. Rohlf
You can also search for this author in PubMed Google Scholar
- D. Sperka
You can also search for this author in PubMed Google Scholar
- L. Sulak
You can also search for this author in PubMed Google Scholar
- S. Bhattacharya
You can also search for this author in PubMed Google Scholar
- D. Cutts
You can also search for this author in PubMed Google Scholar
- A. Ferapontov
You can also search for this author in PubMed Google Scholar
- U. Heintz
You can also search for this author in PubMed Google Scholar
- S. Jabeen
You can also search for this author in PubMed Google Scholar
- G. Kukartsev
You can also search for this author in PubMed Google Scholar
- G. Landsberg
You can also search for this author in PubMed Google Scholar
- M. Luk
You can also search for this author in PubMed Google Scholar
- M. Narain
You can also search for this author in PubMed Google Scholar
- D. Nguyen
You can also search for this author in PubMed Google Scholar
- M. Segala
You can also search for this author in PubMed Google Scholar
- T. Sinthuprasith
You can also search for this author in PubMed Google Scholar
- T. Speer
You can also search for this author in PubMed Google Scholar
- K. V. Tsang
You can also search for this author in PubMed Google Scholar
- R. Breedon
You can also search for this author in PubMed Google Scholar
- G. Breto
You can also search for this author in PubMed Google Scholar
- M. Calderon De La Barca Sanchez
You can also search for this author in PubMed Google Scholar
- M. Caulfield
You can also search for this author in PubMed Google Scholar
- S. Chauhan
You can also search for this author in PubMed Google Scholar
- M. Chertok
You can also search for this author in PubMed Google Scholar
- J. Conway
You can also search for this author in PubMed Google Scholar
- R. Conway
You can also search for this author in PubMed Google Scholar
- P. T. Cox
You can also search for this author in PubMed Google Scholar
- J. Dolen
You can also search for this author in PubMed Google Scholar
- R. Erbacher
You can also search for this author in PubMed Google Scholar
- M. Gardner
You can also search for this author in PubMed Google Scholar
- R. Houtz
You can also search for this author in PubMed Google Scholar
- W. Ko
You can also search for this author in PubMed Google Scholar
- A. Kopecky
You can also search for this author in PubMed Google Scholar
- R. Lander
You can also search for this author in PubMed Google Scholar
- O. Mall
You can also search for this author in PubMed Google Scholar
- T. Miceli
You can also search for this author in PubMed Google Scholar
- R. Nelson
You can also search for this author in PubMed Google Scholar
- D. Pellett
You can also search for this author in PubMed Google Scholar
- J. Robles
You can also search for this author in PubMed Google Scholar
- B. Rutherford
You can also search for this author in PubMed Google Scholar
- M. Searle
You can also search for this author in PubMed Google Scholar
- J. Smith
You can also search for this author in PubMed Google Scholar
- M. Squires
You can also search for this author in PubMed Google Scholar
- M. Tripathi
You can also search for this author in PubMed Google Scholar
- R. Vasquez Sierra
You can also search for this author in PubMed Google Scholar
- V. Andreev
You can also search for this author in PubMed Google Scholar
- K. Arisaka
You can also search for this author in PubMed Google Scholar
- D. Cline
You can also search for this author in PubMed Google Scholar
- R. Cousins
You can also search for this author in PubMed Google Scholar
- J. Duris
You can also search for this author in PubMed Google Scholar
- S. Erhan
You can also search for this author in PubMed Google Scholar
- P. Everaerts
You can also search for this author in PubMed Google Scholar
- C. Farrell
You can also search for this author in PubMed Google Scholar
- J. Hauser
You can also search for this author in PubMed Google Scholar
- M. Ignatenko
You can also search for this author in PubMed Google Scholar
- C. Jarvis
You can also search for this author in PubMed Google Scholar
- C. Plager
You can also search for this author in PubMed Google Scholar
- G. Rakness
You can also search for this author in PubMed Google Scholar
- P. Schlein
You can also search for this author in PubMed Google Scholar
- J. Tucker
You can also search for this author in PubMed Google Scholar
- V. Valuev
You can also search for this author in PubMed Google Scholar
- M. Weber
You can also search for this author in PubMed Google Scholar
- J. Babb
You can also search for this author in PubMed Google Scholar
- R. Clare
You can also search for this author in PubMed Google Scholar
- J. Ellison
You can also search for this author in PubMed Google Scholar
- J. W. Gary
You can also search for this author in PubMed Google Scholar
- F. Giordano
You can also search for this author in PubMed Google Scholar
- G. Hanson
You can also search for this author in PubMed Google Scholar
- G. Y. Jeng
You can also search for this author in PubMed Google Scholar
- H. Liu
You can also search for this author in PubMed Google Scholar
- O. R. Long
You can also search for this author in PubMed Google Scholar
- A. Luthra
You can also search for this author in PubMed Google Scholar
- H. Nguyen
You can also search for this author in PubMed Google Scholar
- S. Paramesvaran
You can also search for this author in PubMed Google Scholar
- J. Sturdy
You can also search for this author in PubMed Google Scholar
- S. Sumowidagdo
You can also search for this author in PubMed Google Scholar
- R. Wilken
You can also search for this author in PubMed Google Scholar
- S. Wimpenny
You can also search for this author in PubMed Google Scholar
- W. Andrews
You can also search for this author in PubMed Google Scholar
- J. G. Branson
You can also search for this author in PubMed Google Scholar
- G. B. Cerati
You can also search for this author in PubMed Google Scholar
- S. Cittolin
You can also search for this author in PubMed Google Scholar
- D. Evans
You can also search for this author in PubMed Google Scholar
- F. Golf
You can also search for this author in PubMed Google Scholar
- A. Holzner
You can also search for this author in PubMed Google Scholar
- R. Kelley
You can also search for this author in PubMed Google Scholar
- M. Lebourgeois
You can also search for this author in PubMed Google Scholar
- J. Letts
You can also search for this author in PubMed Google Scholar
- I. Macneill
You can also search for this author in PubMed Google Scholar
- B. Mangano
You can also search for this author in PubMed Google Scholar
- S. Padhi
You can also search for this author in PubMed Google Scholar
- C. Palmer
You can also search for this author in PubMed Google Scholar
- G. Petrucciani
You can also search for this author in PubMed Google Scholar
- H. Pi
You can also search for this author in PubMed Google Scholar
- M. Pieri
You can also search for this author in PubMed Google Scholar
- R. Ranieri
You can also search for this author in PubMed Google Scholar
- M. Sani
You can also search for this author in PubMed Google Scholar
- I. Sfiligoi
You can also search for this author in PubMed Google Scholar
- V. Sharma
You can also search for this author in PubMed Google Scholar
- S. Simon
You can also search for this author in PubMed Google Scholar
- E. Sudano
You can also search for this author in PubMed Google Scholar
- M. Tadel
You can also search for this author in PubMed Google Scholar
- Y. Tu
You can also search for this author in PubMed Google Scholar
- A. Vartak
You can also search for this author in PubMed Google Scholar
- S. Wasserbaech
You can also search for this author in PubMed Google Scholar
- F. Würthwein
You can also search for this author in PubMed Google Scholar
- A. Yagil
You can also search for this author in PubMed Google Scholar
- J. Yoo
You can also search for this author in PubMed Google Scholar
- D. Barge
You can also search for this author in PubMed Google Scholar
- R. Bellan
You can also search for this author in PubMed Google Scholar
- C. Campagnari
You can also search for this author in PubMed Google Scholar
- M. D’Alfonso
You can also search for this author in PubMed Google Scholar
- T. Danielson
You can also search for this author in PubMed Google Scholar
- K. Flowers
You can also search for this author in PubMed Google Scholar
- P. Geffert
You can also search for this author in PubMed Google Scholar
- J. Incandela
You can also search for this author in PubMed Google Scholar
- C. Justus
You can also search for this author in PubMed Google Scholar
- P. Kalavase
You can also search for this author in PubMed Google Scholar
- S. A. Koay
You can also search for this author in PubMed Google Scholar
- D. Kovalskyi
You can also search for this author in PubMed Google Scholar
- V. Krutelyov
You can also search for this author in PubMed Google Scholar
- S. Lowette
You can also search for this author in PubMed Google Scholar
- N. Mccoll
You can also search for this author in PubMed Google Scholar
- V. Pavlunin
You can also search for this author in PubMed Google Scholar
- F. Rebassoo
You can also search for this author in PubMed Google Scholar
- J. Ribnik
You can also search for this author in PubMed Google Scholar
- J. Richman
You can also search for this author in PubMed Google Scholar
- R. Rossin
You can also search for this author in PubMed Google Scholar
- D. Stuart
You can also search for this author in PubMed Google Scholar
- W. To
You can also search for this author in PubMed Google Scholar
- J. R. Vlimant
You can also search for this author in PubMed Google Scholar
- C. West
You can also search for this author in PubMed Google Scholar
- A. Apresyan
You can also search for this author in PubMed Google Scholar
- A. Bornheim
You can also search for this author in PubMed Google Scholar
- J. Bunn
You can also search for this author in PubMed Google Scholar
- Y. Chen
You can also search for this author in PubMed Google Scholar
- E. Di Marco
You can also search for this author in PubMed Google Scholar
- J. Duarte
You can also search for this author in PubMed Google Scholar
- M. Gataullin
You can also search for this author in PubMed Google Scholar
- Y. Ma
You can also search for this author in PubMed Google Scholar
- A. Mott
You can also search for this author in PubMed Google Scholar
- H. B. Newman
You can also search for this author in PubMed Google Scholar
- C. Rogan
You can also search for this author in PubMed Google Scholar
- V. Timciuc
You can also search for this author in PubMed Google Scholar
- P. Traczyk
You can also search for this author in PubMed Google Scholar
- J. Veverka
You can also search for this author in PubMed Google Scholar
- R. Wilkinson
You can also search for this author in PubMed Google Scholar
- Y. Yang
You can also search for this author in PubMed Google Scholar
- R. Y. Zhu
You can also search for this author in PubMed Google Scholar
- B. Akgun
You can also search for this author in PubMed Google Scholar
- R. Carroll
You can also search for this author in PubMed Google Scholar
- T. Ferguson
You can also search for this author in PubMed Google Scholar
- Y. Iiyama
You can also search for this author in PubMed Google Scholar
- D. W. Jang
You can also search for this author in PubMed Google Scholar
- S. Y. Jun
You can also search for this author in PubMed Google Scholar
- Y. F. Liu
You can also search for this author in PubMed Google Scholar
- M. Paulini
You can also search for this author in PubMed Google Scholar
- J. Russ
You can also search for this author in PubMed Google Scholar
- H. Vogel
You can also search for this author in PubMed Google Scholar
- I. Vorobiev
You can also search for this author in PubMed Google Scholar
- J. P. Cumalat
You can also search for this author in PubMed Google Scholar
- M. E. Dinardo
You can also search for this author in PubMed Google Scholar
- B. R. Drell
You can also search for this author in PubMed Google Scholar
- C. J. Edelmaier
You can also search for this author in PubMed Google Scholar
- W. T. Ford
You can also search for this author in PubMed Google Scholar
- A. Gaz
You can also search for this author in PubMed Google Scholar
- B. Heyburn
You can also search for this author in PubMed Google Scholar
- E. Luiggi Lopez
You can also search for this author in PubMed Google Scholar
- U. Nauenberg
You can also search for this author in PubMed Google Scholar
- J. G. Smith
You can also search for this author in PubMed Google Scholar
- K. Stenson
You can also search for this author in PubMed Google Scholar
- K. A. Ulmer
You can also search for this author in PubMed Google Scholar
- S. R. Wagner
You can also search for this author in PubMed Google Scholar
- S. L. Zang
You can also search for this author in PubMed Google Scholar
- L. Agostino
You can also search for this author in PubMed Google Scholar
- J. Alexander
You can also search for this author in PubMed Google Scholar
- A. Chatterjee
You can also search for this author in PubMed Google Scholar
- N. Eggert
You can also search for this author in PubMed Google Scholar
- L. K. Gibbons
You can also search for this author in PubMed Google Scholar
- B. Heltsley
You can also search for this author in PubMed Google Scholar
- W. Hopkins
You can also search for this author in PubMed Google Scholar
- A. Khukhunaishvili
You can also search for this author in PubMed Google Scholar
- B. Kreis
You can also search for this author in PubMed Google Scholar
- N. Mirman
You can also search for this author in PubMed Google Scholar
- G. Nicolas Kaufman
You can also search for this author in PubMed Google Scholar
- J. R. Patterson
You can also search for this author in PubMed Google Scholar
- A. Ryd
You can also search for this author in PubMed Google Scholar
- E. Salvati
You can also search for this author in PubMed Google Scholar
- W. Sun
You can also search for this author in PubMed Google Scholar
- W. D. Teo
You can also search for this author in PubMed Google Scholar
- J. Thom
You can also search for this author in PubMed Google Scholar
- J. Thompson
You can also search for this author in PubMed Google Scholar
- J. Vaughan
You can also search for this author in PubMed Google Scholar
- Y. Weng
You can also search for this author in PubMed Google Scholar
- L. Winstrom
You can also search for this author in PubMed Google Scholar
- P. Wittich
You can also search for this author in PubMed Google Scholar
- A. Biselli
You can also search for this author in PubMed Google Scholar
- D. Winn
You can also search for this author in PubMed Google Scholar
- S. Abdullin
You can also search for this author in PubMed Google Scholar
- M. Albrow
You can also search for this author in PubMed Google Scholar
- J. Anderson
You can also search for this author in PubMed Google Scholar
- G. Apollinari
You can also search for this author in PubMed Google Scholar
- M. Atac
You can also search for this author in PubMed Google Scholar
- J. A. Bakken
You can also search for this author in PubMed Google Scholar
- L. A. T. Bauerdick
You can also search for this author in PubMed Google Scholar
- A. Beretvas
You can also search for this author in PubMed Google Scholar
- J. Berryhill
You can also search for this author in PubMed Google Scholar
- P. C. Bhat
You can also search for this author in PubMed Google Scholar
- I. Bloch
You can also search for this author in PubMed Google Scholar
- K. Burkett
You can also search for this author in PubMed Google Scholar
- J. N. Butler
You can also search for this author in PubMed Google Scholar
- V. Chetluru
You can also search for this author in PubMed Google Scholar
- H. W. K. Cheung
You can also search for this author in PubMed Google Scholar
- F. Chlebana
You can also search for this author in PubMed Google Scholar
- S. Cihangir
You can also search for this author in PubMed Google Scholar
- W. Cooper
You can also search for this author in PubMed Google Scholar
- D. P. Eartly
You can also search for this author in PubMed Google Scholar
- V. D. Elvira
You can also search for this author in PubMed Google Scholar
- S. Esen
You can also search for this author in PubMed Google Scholar
- I. Fisk
You can also search for this author in PubMed Google Scholar
- J. Freeman
You can also search for this author in PubMed Google Scholar
- Y. Gao
You can also search for this author in PubMed Google Scholar
- E. Gottschalk
You can also search for this author in PubMed Google Scholar
- D. Green
You can also search for this author in PubMed Google Scholar
- O. Gutsche
You can also search for this author in PubMed Google Scholar
- J. Hanlon
You can also search for this author in PubMed Google Scholar
- R. M. Harris
You can also search for this author in PubMed Google Scholar
- J. Hirschauer
You can also search for this author in PubMed Google Scholar
- B. Hooberman
You can also search for this author in PubMed Google Scholar
- H. Jensen
You can also search for this author in PubMed Google Scholar
- S. Jindariani
You can also search for this author in PubMed Google Scholar
- M. Johnson
You can also search for this author in PubMed Google Scholar
- U. Joshi
You can also search for this author in PubMed Google Scholar
- B. Klima
You can also search for this author in PubMed Google Scholar
- S. Kunori
You can also search for this author in PubMed Google Scholar
- S. Kwan
You can also search for this author in PubMed Google Scholar
- C. Leonidopoulos
You can also search for this author in PubMed Google Scholar
- D. Lincoln
You can also search for this author in PubMed Google Scholar
- R. Lipton
You can also search for this author in PubMed Google Scholar
- J. Lykken
You can also search for this author in PubMed Google Scholar
- K. Maeshima
You can also search for this author in PubMed Google Scholar
- J. M. Marraffino
You can also search for this author in PubMed Google Scholar
- S. Maruyama
You can also search for this author in PubMed Google Scholar
- D. Mason
You can also search for this author in PubMed Google Scholar
- P. McBride
You can also search for this author in PubMed Google Scholar
- T. Miao
You can also search for this author in PubMed Google Scholar
- K. Mishra
You can also search for this author in PubMed Google Scholar
- S. Mrenna
You can also search for this author in PubMed Google Scholar
- Y. Musienko
You can also search for this author in PubMed Google Scholar
- C. Newman-Holmes
You can also search for this author in PubMed Google Scholar
- V. O’Dell
You can also search for this author in PubMed Google Scholar
- J. Pivarski
You can also search for this author in PubMed Google Scholar
- R. Pordes
You can also search for this author in PubMed Google Scholar
- O. Prokofyev
You can also search for this author in PubMed Google Scholar
- T. Schwarz
You can also search for this author in PubMed Google Scholar
- E. Sexton-Kennedy
You can also search for this author in PubMed Google Scholar
- S. Sharma
You can also search for this author in PubMed Google Scholar
- W. J. Spalding
You can also search for this author in PubMed Google Scholar
- L. Spiegel
You can also search for this author in PubMed Google Scholar
- P. Tan
You can also search for this author in PubMed Google Scholar
- L. Taylor
You can also search for this author in PubMed Google Scholar
- S. Tkaczyk
You can also search for this author in PubMed Google Scholar
- L. Uplegger
You can also search for this author in PubMed Google Scholar
- E. W. Vaandering
You can also search for this author in PubMed Google Scholar
- R. Vidal
You can also search for this author in PubMed Google Scholar
- J. Whitmore
You can also search for this author in PubMed Google Scholar
- W. Wu
You can also search for this author in PubMed Google Scholar
- F. Yang
You can also search for this author in PubMed Google Scholar
- F. Yumiceva
You can also search for this author in PubMed Google Scholar
- J. C. Yun
You can also search for this author in PubMed Google Scholar
- D. Acosta
You can also search for this author in PubMed Google Scholar
- P. Avery
You can also search for this author in PubMed Google Scholar
- D. Bourilkov
You can also search for this author in PubMed Google Scholar
- M. Chen
You can also search for this author in PubMed Google Scholar
- S. Das
You can also search for this author in PubMed Google Scholar
- M. De Gruttola
You can also search for this author in PubMed Google Scholar
- G. P. Di Giovanni
You can also search for this author in PubMed Google Scholar
- D. Dobur
You can also search for this author in PubMed Google Scholar
- A. Drozdetskiy
You can also search for this author in PubMed Google Scholar
- R. D. Field
You can also search for this author in PubMed Google Scholar
- M. Fisher
You can also search for this author in PubMed Google Scholar
- Y. Fu
You can also search for this author in PubMed Google Scholar
- I. K. Furic
You can also search for this author in PubMed Google Scholar
- J. Gartner
You can also search for this author in PubMed Google Scholar
- S. Goldberg
You can also search for this author in PubMed Google Scholar
- J. Hugon
You can also search for this author in PubMed Google Scholar
- B. Kim
You can also search for this author in PubMed Google Scholar
- J. Konigsberg
You can also search for this author in PubMed Google Scholar
- A. Korytov
You can also search for this author in PubMed Google Scholar
- A. Kropivnitskaya
You can also search for this author in PubMed Google Scholar
- T. Kypreos
You can also search for this author in PubMed Google Scholar
- J. F. Low
You can also search for this author in PubMed Google Scholar
- K. Matchev
You can also search for this author in PubMed Google Scholar
- P. Milenovic
You can also search for this author in PubMed Google Scholar
- G. Mitselmakher
You can also search for this author in PubMed Google Scholar
- L. Muniz
You can also search for this author in PubMed Google Scholar
- R. Remington
You can also search for this author in PubMed Google Scholar
- A. Rinkevicius
You can also search for this author in PubMed Google Scholar
- M. Schmitt
You can also search for this author in PubMed Google Scholar
- B. Scurlock
You can also search for this author in PubMed Google Scholar
- P. Sellers
You can also search for this author in PubMed Google Scholar
- N. Skhirtladze
You can also search for this author in PubMed Google Scholar
- M. Snowball
You can also search for this author in PubMed Google Scholar
- D. Wang
You can also search for this author in PubMed Google Scholar
- J. Yelton
You can also search for this author in PubMed Google Scholar
- M. Zakaria
You can also search for this author in PubMed Google Scholar
- V. Gaultney
You can also search for this author in PubMed Google Scholar
- L. M. Lebolo
You can also search for this author in PubMed Google Scholar
- S. Linn
You can also search for this author in PubMed Google Scholar
- P. Markowitz
You can also search for this author in PubMed Google Scholar
- G. Martinez
You can also search for this author in PubMed Google Scholar
- J. L. Rodriguez
You can also search for this author in PubMed Google Scholar
- T. Adams
You can also search for this author in PubMed Google Scholar
- A. Askew
You can also search for this author in PubMed Google Scholar
- J. Bochenek
You can also search for this author in PubMed Google Scholar
- J. Chen
You can also search for this author in PubMed Google Scholar
- B. Diamond
You can also search for this author in PubMed Google Scholar
- S. V. Gleyzer
You can also search for this author in PubMed Google Scholar
- J. Haas
You can also search for this author in PubMed Google Scholar
- S. Hagopian
You can also search for this author in PubMed Google Scholar
- V. Hagopian
You can also search for this author in PubMed Google Scholar
- M. Jenkins
You can also search for this author in PubMed Google Scholar
- K. F. Johnson
You can also search for this author in PubMed Google Scholar
- H. Prosper
You can also search for this author in PubMed Google Scholar
- S. Sekmen
You can also search for this author in PubMed Google Scholar
- V. Veeraraghavan
You can also search for this author in PubMed Google Scholar
- M. Weinberg
You can also search for this author in PubMed Google Scholar
- M. M. Baarmand
You can also search for this author in PubMed Google Scholar
- B. Dorney
You can also search for this author in PubMed Google Scholar
- M. Hohlmann
You can also search for this author in PubMed Google Scholar
- H. Kalakhety
You can also search for this author in PubMed Google Scholar
- I. Vodopiyanov
You can also search for this author in PubMed Google Scholar
- M. R. Adams
You can also search for this author in PubMed Google Scholar
- I. M. Anghel
You can also search for this author in PubMed Google Scholar
- L. Apanasevich
You can also search for this author in PubMed Google Scholar
- Y. Bai
You can also search for this author in PubMed Google Scholar
- V. E. Bazterra
You can also search for this author in PubMed Google Scholar
- R. R. Betts
You can also search for this author in PubMed Google Scholar
- J. Callner
You can also search for this author in PubMed Google Scholar
- R. Cavanaugh
You can also search for this author in PubMed Google Scholar
- C. Dragoiu
You can also search for this author in PubMed Google Scholar
- L. Gauthier
You can also search for this author in PubMed Google Scholar
- C. E. Gerber
You can also search for this author in PubMed Google Scholar
- D. J. Hofman
You can also search for this author in PubMed Google Scholar
- S. Khalatyan
You can also search for this author in PubMed Google Scholar
- G. J. Kunde
You can also search for this author in PubMed Google Scholar
- F. Lacroix
You can also search for this author in PubMed Google Scholar
- M. Malek
You can also search for this author in PubMed Google Scholar
- C. O’Brien
You can also search for this author in PubMed Google Scholar
- C. Silkworth
You can also search for this author in PubMed Google Scholar
- C. Silvestre
You can also search for this author in PubMed Google Scholar
- D. Strom
You can also search for this author in PubMed Google Scholar
- N. Varelas
You can also search for this author in PubMed Google Scholar
- U. Akgun
You can also search for this author in PubMed Google Scholar
- E. A. Albayrak
You can also search for this author in PubMed Google Scholar
- B. Bilki
You can also search for this author in PubMed Google Scholar
- W. Clarida
You can also search for this author in PubMed Google Scholar
- F. Duru
You can also search for this author in PubMed Google Scholar
- S. Griffiths
You can also search for this author in PubMed Google Scholar
- C. K. Lae
You can also search for this author in PubMed Google Scholar
- E. McCliment
You can also search for this author in PubMed Google Scholar
- J.-P. Merlo
You can also search for this author in PubMed Google Scholar
- H. Mermerkaya
You can also search for this author in PubMed Google Scholar
- A. Mestvirishvili
You can also search for this author in PubMed Google Scholar
- A. Moeller
You can also search for this author in PubMed Google Scholar
- J. Nachtman
You can also search for this author in PubMed Google Scholar
- C. R. Newsom
You can also search for this author in PubMed Google Scholar
- E. Norbeck
You can also search for this author in PubMed Google Scholar
- J. Olson
You can also search for this author in PubMed Google Scholar
- Y. Onel
You can also search for this author in PubMed Google Scholar
- F. Ozok
You can also search for this author in PubMed Google Scholar
- S. Sen
You can also search for this author in PubMed Google Scholar
- E. Tiras
You can also search for this author in PubMed Google Scholar
- J. Wetzel
You can also search for this author in PubMed Google Scholar
- T. Yetkin
You can also search for this author in PubMed Google Scholar
- K. Yi
You can also search for this author in PubMed Google Scholar
- B. A. Barnett
You can also search for this author in PubMed Google Scholar
- B. Blumenfeld
You can also search for this author in PubMed Google Scholar
- S. Bolognesi
You can also search for this author in PubMed Google Scholar
- A. Bonato
You can also search for this author in PubMed Google Scholar
- D. Fehling
You can also search for this author in PubMed Google Scholar
- G. Giurgiu
You can also search for this author in PubMed Google Scholar
- A. V. Gritsan
You can also search for this author in PubMed Google Scholar
- Z. J. Guo
You can also search for this author in PubMed Google Scholar
- G. Hu
You can also search for this author in PubMed Google Scholar
- P. Maksimovic
You can also search for this author in PubMed Google Scholar
- S. Rappoccio
You can also search for this author in PubMed Google Scholar
- M. Swartz
You can also search for this author in PubMed Google Scholar
- N. V. Tran
You can also search for this author in PubMed Google Scholar
- A. Whitbeck
You can also search for this author in PubMed Google Scholar
- P. Baringer
You can also search for this author in PubMed Google Scholar
- A. Bean
You can also search for this author in PubMed Google Scholar
- G. Benelli
You can also search for this author in PubMed Google Scholar
- O. Grachov
You can also search for this author in PubMed Google Scholar
- R. P. Kenny Iii
You can also search for this author in PubMed Google Scholar
- M. Murray
You can also search for this author in PubMed Google Scholar
- D. Noonan
You can also search for this author in PubMed Google Scholar
- S. Sanders
You can also search for this author in PubMed Google Scholar
- R. Stringer
You can also search for this author in PubMed Google Scholar
- G. Tinti
You can also search for this author in PubMed Google Scholar
- J. S. Wood
You can also search for this author in PubMed Google Scholar
- V. Zhukova
You can also search for this author in PubMed Google Scholar
- A. F. Barfuss
You can also search for this author in PubMed Google Scholar
- T. Bolton
You can also search for this author in PubMed Google Scholar
- I. Chakaberia
You can also search for this author in PubMed Google Scholar
- A. Ivanov
You can also search for this author in PubMed Google Scholar
- S. Khalil
You can also search for this author in PubMed Google Scholar
- M. Makouski
You can also search for this author in PubMed Google Scholar
- Y. Maravin
You can also search for this author in PubMed Google Scholar
- S. Shrestha
You can also search for this author in PubMed Google Scholar
- I. Svintradze
You can also search for this author in PubMed Google Scholar
- J. Gronberg
You can also search for this author in PubMed Google Scholar
- D. Lange
You can also search for this author in PubMed Google Scholar
- D. Wright
You can also search for this author in PubMed Google Scholar
- A. Baden
You can also search for this author in PubMed Google Scholar
- M. Boutemeur
You can also search for this author in PubMed Google Scholar
- B. Calvert
You can also search for this author in PubMed Google Scholar
- S. C. Eno
You can also search for this author in PubMed Google Scholar
- J. A. Gomez
You can also search for this author in PubMed Google Scholar
- N. J. Hadley
You can also search for this author in PubMed Google Scholar
- R. G. Kellogg
You can also search for this author in PubMed Google Scholar
- M. Kirn
You can also search for this author in PubMed Google Scholar
- T. Kolberg
You can also search for this author in PubMed Google Scholar
- Y. Lu
You can also search for this author in PubMed Google Scholar
- M. Marionneau
You can also search for this author in PubMed Google Scholar
- A. C. Mignerey
You can also search for this author in PubMed Google Scholar
- A. Peterman
You can also search for this author in PubMed Google Scholar
- K. Rossato
You can also search for this author in PubMed Google Scholar
- P. Rumerio
You can also search for this author in PubMed Google Scholar
- A. Skuja
You can also search for this author in PubMed Google Scholar
- J. Temple
You can also search for this author in PubMed Google Scholar
- M. B. Tonjes
You can also search for this author in PubMed Google Scholar
- S. C. Tonwar
You can also search for this author in PubMed Google Scholar
- E. Twedt
You can also search for this author in PubMed Google Scholar
- B. Alver
You can also search for this author in PubMed Google Scholar
- G. Bauer
You can also search for this author in PubMed Google Scholar
- J. Bendavid
You can also search for this author in PubMed Google Scholar
- W. Busza
You can also search for this author in PubMed Google Scholar
- E. Butz
You can also search for this author in PubMed Google Scholar
- I. A. Cali
You can also search for this author in PubMed Google Scholar
- M. Chan
You can also search for this author in PubMed Google Scholar
- V. Dutta
You can also search for this author in PubMed Google Scholar
- G. Gomez Ceballos
You can also search for this author in PubMed Google Scholar
- M. Goncharov
You can also search for this author in PubMed Google Scholar
- K. A. Hahn
You can also search for this author in PubMed Google Scholar
- Y. Kim
You can also search for this author in PubMed Google Scholar
- M. Klute
You can also search for this author in PubMed Google Scholar
- Y.-J. Lee
You can also search for this author in PubMed Google Scholar
- W. Li
You can also search for this author in PubMed Google Scholar
- P. D. Luckey
You can also search for this author in PubMed Google Scholar
- T. Ma
You can also search for this author in PubMed Google Scholar
- S. Nahn
You can also search for this author in PubMed Google Scholar
- C. Paus
You can also search for this author in PubMed Google Scholar
- D. Ralph
You can also search for this author in PubMed Google Scholar
- C. Roland
You can also search for this author in PubMed Google Scholar
- G. Roland
You can also search for this author in PubMed Google Scholar
- M. Rudolph
You can also search for this author in PubMed Google Scholar
- G. S. F. Stephans
You can also search for this author in PubMed Google Scholar
- F. Stöckli
You can also search for this author in PubMed Google Scholar
- K. Sumorok
You can also search for this author in PubMed Google Scholar
- K. Sung
You can also search for this author in PubMed Google Scholar
- D. Velicanu
You can also search for this author in PubMed Google Scholar
- E. A. Wenger
You can also search for this author in PubMed Google Scholar
- R. Wolf
You can also search for this author in PubMed Google Scholar
- B. Wyslouch
You can also search for this author in PubMed Google Scholar
- S. Xie
You can also search for this author in PubMed Google Scholar
- M. Yang
You can also search for this author in PubMed Google Scholar
- Y. Yilmaz
You can also search for this author in PubMed Google Scholar
- A. S. Yoon
You can also search for this author in PubMed Google Scholar
- M. Zanetti
You can also search for this author in PubMed Google Scholar
- S. I. Cooper
You can also search for this author in PubMed Google Scholar
- P. Cushman
You can also search for this author in PubMed Google Scholar
- B. Dahmes
You can also search for this author in PubMed Google Scholar
- A. De Benedetti
You can also search for this author in PubMed Google Scholar
- G. Franzoni
You can also search for this author in PubMed Google Scholar
- A. Gude
You can also search for this author in PubMed Google Scholar
- J. Haupt
You can also search for this author in PubMed Google Scholar
- S. C. Kao
You can also search for this author in PubMed Google Scholar
- K. Klapoetke
You can also search for this author in PubMed Google Scholar
- Y. Kubota
You can also search for this author in PubMed Google Scholar
- J. Mans
You can also search for this author in PubMed Google Scholar
- N. Pastika
You can also search for this author in PubMed Google Scholar
- V. Rekovic
You can also search for this author in PubMed Google Scholar
- R. Rusack
You can also search for this author in PubMed Google Scholar
- M. Sasseville
You can also search for this author in PubMed Google Scholar
- A. Singovsky
You can also search for this author in PubMed Google Scholar
- N. Tambe
You can also search for this author in PubMed Google Scholar
- J. Turkewitz
You can also search for this author in PubMed Google Scholar
- L. M. Cremaldi
You can also search for this author in PubMed Google Scholar
- R. Godang
You can also search for this author in PubMed Google Scholar
- R. Kroeger
You can also search for this author in PubMed Google Scholar
- L. Perera
You can also search for this author in PubMed Google Scholar
- R. Rahmat
You can also search for this author in PubMed Google Scholar
- D. A. Sanders
You can also search for this author in PubMed Google Scholar
- D. Summers
You can also search for this author in PubMed Google Scholar
- E. Avdeeva
You can also search for this author in PubMed Google Scholar
- K. Bloom
You can also search for this author in PubMed Google Scholar
- S. Bose
You can also search for this author in PubMed Google Scholar
- J. Butt
You can also search for this author in PubMed Google Scholar
- D. R. Claes
You can also search for this author in PubMed Google Scholar
- A. Dominguez
You can also search for this author in PubMed Google Scholar
- M. Eads
You can also search for this author in PubMed Google Scholar
- P. Jindal
You can also search for this author in PubMed Google Scholar
- J. Keller
You can also search for this author in PubMed Google Scholar
- I. Kravchenko
You can also search for this author in PubMed Google Scholar
- J. Lazo-Flores
You can also search for this author in PubMed Google Scholar
- H. Malbouisson
You can also search for this author in PubMed Google Scholar
- S. Malik
You can also search for this author in PubMed Google Scholar
- G. R. Snow
You can also search for this author in PubMed Google Scholar
- U. Baur
You can also search for this author in PubMed Google Scholar
- A. Godshalk
You can also search for this author in PubMed Google Scholar
- I. Iashvili
You can also search for this author in PubMed Google Scholar
- S. Jain
You can also search for this author in PubMed Google Scholar
- A. Kharchilava
You can also search for this author in PubMed Google Scholar
- A. Kumar
You can also search for this author in PubMed Google Scholar
- S. P. Shipkowski
You can also search for this author in PubMed Google Scholar
- K. Smith
You can also search for this author in PubMed Google Scholar
- Z. Wan
You can also search for this author in PubMed Google Scholar
- G. Alverson
You can also search for this author in PubMed Google Scholar
- E. Barberis
You can also search for this author in PubMed Google Scholar
- D. Baumgartel
You can also search for this author in PubMed Google Scholar
- M. Chasco
You can also search for this author in PubMed Google Scholar
- D. Trocino
You can also search for this author in PubMed Google Scholar
- D. Wood
You can also search for this author in PubMed Google Scholar
- J. Zhang
You can also search for this author in PubMed Google Scholar
- A. Anastassov
You can also search for this author in PubMed Google Scholar
- A. Kubik
You can also search for this author in PubMed Google Scholar
- N. Mucia
You can also search for this author in PubMed Google Scholar
- N. Odell
You can also search for this author in PubMed Google Scholar
- R. A. Ofierzynski
You can also search for this author in PubMed Google Scholar
- B. Pollack
You can also search for this author in PubMed Google Scholar
- A. Pozdnyakov
You can also search for this author in PubMed Google Scholar
- M. Schmitt
You can also search for this author in PubMed Google Scholar
- S. Stoynev
You can also search for this author in PubMed Google Scholar
- M. Velasco
You can also search for this author in PubMed Google Scholar
- S. Won
You can also search for this author in PubMed Google Scholar
- L. Antonelli
You can also search for this author in PubMed Google Scholar
- D. Berry
You can also search for this author in PubMed Google Scholar
- A. Brinkerhoff
You can also search for this author in PubMed Google Scholar
- M. Hildreth
You can also search for this author in PubMed Google Scholar
- C. Jessop
You can also search for this author in PubMed Google Scholar
- D. J. Karmgard
You can also search for this author in PubMed Google Scholar
- J. Kolb
You can also search for this author in PubMed Google Scholar
- K. Lannon
You can also search for this author in PubMed Google Scholar
- W. Luo
You can also search for this author in PubMed Google Scholar
- S. Lynch
You can also search for this author in PubMed Google Scholar
- N. Marinelli
You can also search for this author in PubMed Google Scholar
- D. M. Morse
You can also search for this author in PubMed Google Scholar
- T. Pearson
You can also search for this author in PubMed Google Scholar
- R. Ruchti
You can also search for this author in PubMed Google Scholar
- J. Slaunwhite
You can also search for this author in PubMed Google Scholar
- N. Valls
You can also search for this author in PubMed Google Scholar
- M. Wayne
You can also search for this author in PubMed Google Scholar
- M. Wolf
You can also search for this author in PubMed Google Scholar
- J. Ziegler
You can also search for this author in PubMed Google Scholar
- B. Bylsma
You can also search for this author in PubMed Google Scholar
- L. S. Durkin
You can also search for this author in PubMed Google Scholar
- C. Hill
You can also search for this author in PubMed Google Scholar
- P. Killewald
You can also search for this author in PubMed Google Scholar
- K. Kotov
You can also search for this author in PubMed Google Scholar
- T. Y. Ling
You can also search for this author in PubMed Google Scholar
- D. Puigh
You can also search for this author in PubMed Google Scholar
- M. Rodenburg
You can also search for this author in PubMed Google Scholar
- C. Vuosalo
You can also search for this author in PubMed Google Scholar
- G. Williams
You can also search for this author in PubMed Google Scholar
- N. Adam
You can also search for this author in PubMed Google Scholar
- E. Berry
You can also search for this author in PubMed Google Scholar
- P. Elmer
You can also search for this author in PubMed Google Scholar
- D. Gerbaudo
You can also search for this author in PubMed Google Scholar
- V. Halyo
You can also search for this author in PubMed Google Scholar
- P. Hebda
You can also search for this author in PubMed Google Scholar
- J. Hegeman
You can also search for this author in PubMed Google Scholar
- A. Hunt
You can also search for this author in PubMed Google Scholar
- E. Laird
You can also search for this author in PubMed Google Scholar
- D. Lopes Pegna
You can also search for this author in PubMed Google Scholar
- P. Lujan
You can also search for this author in PubMed Google Scholar
- D. Marlow
You can also search for this author in PubMed Google Scholar
- T. Medvedeva
You can also search for this author in PubMed Google Scholar
- M. Mooney
You can also search for this author in PubMed Google Scholar
- J. Olsen
You can also search for this author in PubMed Google Scholar
- P. Piroué
You can also search for this author in PubMed Google Scholar
- X. Quan
You can also search for this author in PubMed Google Scholar
- A. Raval
You can also search for this author in PubMed Google Scholar
- H. Saka
You can also search for this author in PubMed Google Scholar
- D. Stickland
You can also search for this author in PubMed Google Scholar
- C. Tully
You can also search for this author in PubMed Google Scholar
- J. S. Werner
You can also search for this author in PubMed Google Scholar
- A. Zuranski
You can also search for this author in PubMed Google Scholar
- J. G. Acosta
You can also search for this author in PubMed Google Scholar
- X. T. Huang
You can also search for this author in PubMed Google Scholar
- A. Lopez
You can also search for this author in PubMed Google Scholar
- H. Mendez
You can also search for this author in PubMed Google Scholar
- S. Oliveros
You can also search for this author in PubMed Google Scholar
- J. E. Ramirez Vargas
You can also search for this author in PubMed Google Scholar
- A. Zatserklyaniy
You can also search for this author in PubMed Google Scholar
- E. Alagoz
You can also search for this author in PubMed Google Scholar
- V. E. Barnes
You can also search for this author in PubMed Google Scholar
- D. Benedetti
You can also search for this author in PubMed Google Scholar
- G. Bolla
You can also search for this author in PubMed Google Scholar
- D. Bortoletto
You can also search for this author in PubMed Google Scholar
- M. De Mattia
You can also search for this author in PubMed Google Scholar
- A. Everett
You can also search for this author in PubMed Google Scholar
- L. Gutay
You can also search for this author in PubMed Google Scholar
- Z. Hu
You can also search for this author in PubMed Google Scholar
- M. Jones
You can also search for this author in PubMed Google Scholar
- O. Koybasi
You can also search for this author in PubMed Google Scholar
- M. Kress
You can also search for this author in PubMed Google Scholar
- A. T. Laasanen
You can also search for this author in PubMed Google Scholar
- N. Leonardo
You can also search for this author in PubMed Google Scholar
- V. Maroussov
You can also search for this author in PubMed Google Scholar
- P. Merkel
You can also search for this author in PubMed Google Scholar
- D. H. Miller
You can also search for this author in PubMed Google Scholar
- N. Neumeister
You can also search for this author in PubMed Google Scholar
- I. Shipsey
You can also search for this author in PubMed Google Scholar
- D. Silvers
You can also search for this author in PubMed Google Scholar
- A. Svyatkovskiy
You can also search for this author in PubMed Google Scholar
- M. Vidal Marono
You can also search for this author in PubMed Google Scholar
- H. D. Yoo
You can also search for this author in PubMed Google Scholar
- J. Zablocki
You can also search for this author in PubMed Google Scholar
- Y. Zheng
You can also search for this author in PubMed Google Scholar
- S. Guragain
You can also search for this author in PubMed Google Scholar
- N. Parashar
You can also search for this author in PubMed Google Scholar
- A. Adair
You can also search for this author in PubMed Google Scholar
- C. Boulahouache
You can also search for this author in PubMed Google Scholar
- V. Cuplov
You can also search for this author in PubMed Google Scholar
- K. M. Ecklund
You can also search for this author in PubMed Google Scholar
- F. J. M. Geurts
You can also search for this author in PubMed Google Scholar
- B. P. Padley
You can also search for this author in PubMed Google Scholar
- R. Redjimi
You can also search for this author in PubMed Google Scholar
- J. Roberts
You can also search for this author in PubMed Google Scholar
- J. Zabel
You can also search for this author in PubMed Google Scholar
- B. Betchart
You can also search for this author in PubMed Google Scholar
- A. Bodek
You can also search for this author in PubMed Google Scholar
- Y. S. Chung
You can also search for this author in PubMed Google Scholar
- R. Covarelli
You can also search for this author in PubMed Google Scholar
- P. de Barbaro
You can also search for this author in PubMed Google Scholar
- R. Demina
You can also search for this author in PubMed Google Scholar
- Y. Eshaq
You can also search for this author in PubMed Google Scholar
- A. Garcia-Bellido
You can also search for this author in PubMed Google Scholar
- P. Goldenzweig
You can also search for this author in PubMed Google Scholar
- Y. Gotra
You can also search for this author in PubMed Google Scholar
- J. Han
You can also search for this author in PubMed Google Scholar
- A. Harel
You can also search for this author in PubMed Google Scholar
- D. C. Miner
You can also search for this author in PubMed Google Scholar
- G. Petrillo
You can also search for this author in PubMed Google Scholar
- W. Sakumoto
You can also search for this author in PubMed Google Scholar
- D. Vishnevskiy
You can also search for this author in PubMed Google Scholar
- M. Zielinski
You can also search for this author in PubMed Google Scholar
- A. Bhatti
You can also search for this author in PubMed Google Scholar
- R. Ciesielski
You can also search for this author in PubMed Google Scholar
- L. Demortier
You can also search for this author in PubMed Google Scholar
- K. Goulianos
You can also search for this author in PubMed Google Scholar
- G. Lungu
You can also search for this author in PubMed Google Scholar
- S. Malik
You can also search for this author in PubMed Google Scholar
- C. Mesropian
You can also search for this author in PubMed Google Scholar
- S. Arora
You can also search for this author in PubMed Google Scholar
- O. Atramentov
You can also search for this author in PubMed Google Scholar
- A. Barker
You can also search for this author in PubMed Google Scholar
- J. P. Chou
You can also search for this author in PubMed Google Scholar
- C. Contreras-Campana
You can also search for this author in PubMed Google Scholar
- E. Contreras-Campana
You can also search for this author in PubMed Google Scholar
- D. Duggan
You can also search for this author in PubMed Google Scholar
- D. Ferencek
You can also search for this author in PubMed Google Scholar
- Y. Gershtein
You can also search for this author in PubMed Google Scholar
- R. Gray
You can also search for this author in PubMed Google Scholar
- E. Halkiadakis
You can also search for this author in PubMed Google Scholar
- D. Hidas
You can also search for this author in PubMed Google Scholar
- D. Hits
You can also search for this author in PubMed Google Scholar
- A. Lath
You can also search for this author in PubMed Google Scholar
- S. Panwalkar
You can also search for this author in PubMed Google Scholar
- M. Park
You can also search for this author in PubMed Google Scholar
- R. Patel
You can also search for this author in PubMed Google Scholar
- A. Richards
You can also search for this author in PubMed Google Scholar
- K. Rose
You can also search for this author in PubMed Google Scholar
- S. Salur
You can also search for this author in PubMed Google Scholar
- S. Schnetzer
You can also search for this author in PubMed Google Scholar
- C. Seitz
You can also search for this author in PubMed Google Scholar
- S. Somalwar
You can also search for this author in PubMed Google Scholar
- R. Stone
You can also search for this author in PubMed Google Scholar
- S. Thomas
You can also search for this author in PubMed Google Scholar
- G. Cerizza
You can also search for this author in PubMed Google Scholar
- M. Hollingsworth
You can also search for this author in PubMed Google Scholar
- S. Spanier
You can also search for this author in PubMed Google Scholar
- Z. C. Yang
You can also search for this author in PubMed Google Scholar
- A. York
You can also search for this author in PubMed Google Scholar
- R. Eusebi
You can also search for this author in PubMed Google Scholar
- W. Flanagan
You can also search for this author in PubMed Google Scholar
- J. Gilmore
You can also search for this author in PubMed Google Scholar
- T. Kamon
You can also search for this author in PubMed Google Scholar
- V. Khotilovich
You can also search for this author in PubMed Google Scholar
- R. Montalvo
You can also search for this author in PubMed Google Scholar
- I. Osipenkov
You can also search for this author in PubMed Google Scholar
- Y. Pakhotin
You can also search for this author in PubMed Google Scholar
- A. Perloff
You can also search for this author in PubMed Google Scholar
- J. Roe
You can also search for this author in PubMed Google Scholar
- A. Safonov
You can also search for this author in PubMed Google Scholar
- T. Sakuma
You can also search for this author in PubMed Google Scholar
- S. Sengupta
You can also search for this author in PubMed Google Scholar
- I. Suarez
You can also search for this author in PubMed Google Scholar
- A. Tatarinov
You can also search for this author in PubMed Google Scholar
- D. Toback
You can also search for this author in PubMed Google Scholar
- N. Akchurin
You can also search for this author in PubMed Google Scholar
- C. Bardak
You can also search for this author in PubMed Google Scholar
- J. Damgov
You can also search for this author in PubMed Google Scholar
- P. R. Dudero
You can also search for this author in PubMed Google Scholar
- C. Jeong
You can also search for this author in PubMed Google Scholar
- K. Kovitanggoon
You can also search for this author in PubMed Google Scholar
- S. W. Lee
You can also search for this author in PubMed Google Scholar
- T. Libeiro
You can also search for this author in PubMed Google Scholar
- P. Mane
You can also search for this author in PubMed Google Scholar
- Y. Roh
You can also search for this author in PubMed Google Scholar
- A. Sill
You can also search for this author in PubMed Google Scholar
- I. Volobouev
You can also search for this author in PubMed Google Scholar
- R. Wigmans
You can also search for this author in PubMed Google Scholar
- E. Appelt
You can also search for this author in PubMed Google Scholar
- E. Brownson
You can also search for this author in PubMed Google Scholar
- D. Engh
You can also search for this author in PubMed Google Scholar
- C. Florez
You can also search for this author in PubMed Google Scholar
- W. Gabella
You can also search for this author in PubMed Google Scholar
- A. Gurrola
You can also search for this author in PubMed Google Scholar
- M. Issah
You can also search for this author in PubMed Google Scholar
- W. Johns
You can also search for this author in PubMed Google Scholar
- P. Kurt
You can also search for this author in PubMed Google Scholar
- C. Maguire
You can also search for this author in PubMed Google Scholar
- A. Melo
You can also search for this author in PubMed Google Scholar
- P. Sheldon
You can also search for this author in PubMed Google Scholar
- B. Snook
You can also search for this author in PubMed Google Scholar
- S. Tuo
You can also search for this author in PubMed Google Scholar
- J. Velkovska
You can also search for this author in PubMed Google Scholar
- M. W. Arenton
You can also search for this author in PubMed Google Scholar
- M. Balazs
You can also search for this author in PubMed Google Scholar
- S. Boutle
You can also search for this author in PubMed Google Scholar
- S. Conetti
You can also search for this author in PubMed Google Scholar
- B. Cox
You can also search for this author in PubMed Google Scholar
- B. Francis
You can also search for this author in PubMed Google Scholar
- S. Goadhouse
You can also search for this author in PubMed Google Scholar
- J. Goodell
You can also search for this author in PubMed Google Scholar
- R. Hirosky
You can also search for this author in PubMed Google Scholar
- A. Ledovskoy
You can also search for this author in PubMed Google Scholar
- C. Lin
You can also search for this author in PubMed Google Scholar
- C. Neu
You can also search for this author in PubMed Google Scholar
- J. Wood
You can also search for this author in PubMed Google Scholar
- R. Yohay
You can also search for this author in PubMed Google Scholar
- S. Gollapinni
You can also search for this author in PubMed Google Scholar
- R. Harr
You can also search for this author in PubMed Google Scholar
- P. E. Karchin
You can also search for this author in PubMed Google Scholar
- C. Kottachchi Kankanamge Don
You can also search for this author in PubMed Google Scholar
- P. Lamichhane
You can also search for this author in PubMed Google Scholar
- M. Mattson
You can also search for this author in PubMed Google Scholar
- C. Milstène
You can also search for this author in PubMed Google Scholar
- A. Sakharov
You can also search for this author in PubMed Google Scholar
- M. Anderson
You can also search for this author in PubMed Google Scholar
- M. Bachtis
You can also search for this author in PubMed Google Scholar
- D. Belknap
You can also search for this author in PubMed Google Scholar
- J. N. Bellinger
You can also search for this author in PubMed Google Scholar
- J. Bernardini
You can also search for this author in PubMed Google Scholar
- L. Borrello
You can also search for this author in PubMed Google Scholar
- D. Carlsmith
You can also search for this author in PubMed Google Scholar
- M. Cepeda
You can also search for this author in PubMed Google Scholar
- S. Dasu
You can also search for this author in PubMed Google Scholar
- J. Efron
You can also search for this author in PubMed Google Scholar
- E. Friis
You can also search for this author in PubMed Google Scholar
- L. Gray
You can also search for this author in PubMed Google Scholar
- K. S. Grogg
You can also search for this author in PubMed Google Scholar
- M. Grothe
You can also search for this author in PubMed Google Scholar
- R. Hall-Wilton
You can also search for this author in PubMed Google Scholar
- M. Herndon
You can also search for this author in PubMed Google Scholar
- A. Hervé
You can also search for this author in PubMed Google Scholar
- P. Klabbers
You can also search for this author in PubMed Google Scholar
- J. Klukas
You can also search for this author in PubMed Google Scholar
- A. Lanaro
You can also search for this author in PubMed Google Scholar
- C. Lazaridis
You can also search for this author in PubMed Google Scholar
- J. Leonard
You can also search for this author in PubMed Google Scholar
- R. Loveless
You can also search for this author in PubMed Google Scholar
- A. Mohapatra
You can also search for this author in PubMed Google Scholar
- I. Ojalvo
You can also search for this author in PubMed Google Scholar
- G. A. Pierro
You can also search for this author in PubMed Google Scholar
- I. Ross
You can also search for this author in PubMed Google Scholar
- A. Savin
You can also search for this author in PubMed Google Scholar
- W. H. Smith
You can also search for this author in PubMed Google Scholar
- J. Swanson
You can also search for this author in PubMed Google Scholar
Consortia
The CMS Collaboration
Rights and permissions
This article is published under an open access license. Please check the 'Copyright Information' section either on this page or in the PDF for details of this license and what re-use is permitted. If your intended use exceeds what is permitted by the license or if you are unable to locate the licence and re-use information, please contact the Rights and Permissions team.
About this article
Cite this article
The CMS Collaboration., Chatrchyan, S., Khachatryan, V. et al. Ratios of dijet production cross sections as a function of the absolute difference in rapidity between jets in proton–proton collisions at \(\sqrt{s} = 7\ \mathrm{TeV}\). Eur. Phys. J. C 72, 2216 (2012). https://doi.org/10.1140/epjc/s10052-012-2216-6
Received:
Revised:
Published:
DOI: https://doi.org/10.1140/epjc/s10052-012-2216-6
Share this article
Anyone you share the following link with will be able to read this content:
Sorry, a shareable link is not currently available for this article.
Provided by the Springer Nature SharedIt content-sharing initiative