Abstract
Raphidioptera, the group of snakeflies, is a rather species-poor in-group of Holometabola. Yet, fossils of snakeflies indicate that the group was more diverse in the past. Here we compare the morphological diversity of snakefly larvae over time. Snakefly larvae are well represented in Cretaceous and Eocene ambers facilitating such a comparison. We used measurements of discrete dimensions as a basis for comparison. This reveals a larger diversity of snakefly larvae in the Cretaceous, especially in relation to head shapes and morphology of the antennae, which were much more variable. In particular, some Cretaceous larvae possessed greatly elongated head capsules and uniquely long and prominent antennae, unparalleled among modern forms. Already by the Eocene, snakefly larvae were less variable than those of the Cretaceous, although some still possessed longer antennae than modern-day larvae. The loss of morphological diversity supports the already well-established loss of taxonomic diversity in the group across time. Quite likely, this also indicates a loss of ecological diversity. These results are comparable to losses in different lineages of the closely related group Neuroptera.
Similar content being viewed by others
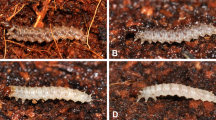
Introduction
Among insects, it is the holometabolans that dominate in terms of species diversity. Specifically, four lineages of Holometabola—Hymenoptera (wasps, bees, ants), Coleoptera (beetles, weevils), Lepidoptera (moths, butterflies), Diptera (mosquitoes, flies, gnats, midges)—together sum up to more than half a million species and comprise the major proportion of terrestrial animal life (e.g., Grimaldi and Engel 2005: Table 1.1. and Fig. 1.6). One aspect of holometabolan success is generally attributed to a niche differentiation between the adult and the morphologically divergent larval forms (e.g., Yang 2001). As many holometabolan species spend a longer proportion of their life as larvae rather than as adults, the true diversity of the group is reflected in their varied larval forms.
Not all holometabolan in-groups are overwhelmingly species-diverse. In fact, a number of biologically interesting groups comprise fewer than 1000 species each. One such small, well-delineated group is Raphidioptera, its representatives commonly known as snakeflies. Snakeflies retain many plesiomorphies as adults, such as a prominent ovipositor in females. In addition, the larvae appear to have a rather plesiomorphic overall morphology. Unlike the larvae of closely related groups, such as Megaloptera or Neuroptera, snakefly larvae lack highly specialised structures such as gills or venom-injecting stylets (e.g., MacLeod 1964; Aspöck and Aspöck 1999, 2007). The overall body of the larvae is rather elongate, with the prognathous head and first thorax dorsum (pronotum) well sclerotised. The antennae are rather short and thin in modern forms, although this varies noticeably among extinct lineages, and the mandibles are typically tough and project forward. The remaining thorax segments and those of the abdomen lack any lateral processes (neither gills nor prolegs or analogous structures). As larvae, snakeflies are generalist predators on small euarthropods and live in the crevices of bark or stones, or in some cases amid detritus on the forest floor (Grimaldi and Engel 2005). The number of larval instars varies among the species, ranging up to as many as ten, and individuals may remain as larvae for several years before pupating. In modern species, pupation is at least partially triggered by extended exposure to cold temperatures. Quite remarkably, snakefly pupae are exarate and active (Grimaldi and Engel 2005; Engel et al. 2018).
There are about 250 modern species of snakeflies confined to the Northern Hemisphere in temperate climates or at higher elevations (Engel et al. 2018). The group was likely much more diverse and widespread in the past, with the modern fauna merely relict (Engel 1995: 187; Aspöck 2002: 35, 36; Aspöck and Aspöck 1999: 24, 2007: 478–480, 2009: 53; Liu et al. 2016; Engel et al. 2018). Snakeflies were most likely part of the first large diversification of the group Holometabola (Aspöck and Aspöck 1999: 24, 2007: 468; Grimaldi and Engel 2005: 335; Pérez-de la Fuente et al. 2012a: 2), fulfilling a number of ecological functions not performed by modern snakeflies.
The fossilized remains of snakefly larvae have been reported from varied deposits, mostly preserved as inclusions in amber. Specimens in Eocene Baltic amber greatly resemble their modern counterparts, while those from Cretaceous ambers appear quite different in certain aspects. Even from the few specimens already documented, the overall head shapes of fossil snakefly larvae were more variable (e.g., Engel 2002; Perrichot and Engel 2007). More distinctively, some Cretaceous specimens differ from modern larvae by their more prominent elongate antennae (Engel 2002; Haug et al. 2020a). These initial observations provided a qualitative indication that the morphological diversity (≈ disparity) among larval snakeflies was greater in the past, and that this may also be a reflection of a once higher species diversity (although greater disparity can also result from fewer, more morphologically divergent species). For the closely related group Neuroptera, a similar qualitative indication (e.g. Wang et al. 2016) was later supported by morphometric quantitative approaches (Haug et al. 2020b, 2022).
Here we report numerous new specimens of larval snakeflies preserved in Eocene and Cretaceous ambers. Furthermore, we explore the morphological disparity and diversity of these larvae by quantitative morphometric methods, comparing three different slices of time (Cretaceous, Eocene, extant) to detect possible changes over the last 100 million years.
Materials and methods
Materials
Four extant specimens of immature snakeflies were directly documented. The specimens originate from the entomological collections of the Centrum für Naturkunde (CeNak), Leibniz-Institut zur Analyse des Biodiversitätswandels (LIB), Hamburg. The species was not pre-determined—the label contained only the year of collection, 1938—the specimens stored together in one jar implies that all four specimens were considered to be conspecific.
Seven snakefly larvae preserved in Eocene Baltic amber were loaned and documented. One specimen comes from the geological–palaeontological collections of the Centrum für Naturkunde (CeNak), Leibniz-Institut zur Analyse des Biodiversitätswandels (LIB), Hamburg (GPIH), one specimen comes from the Geoscience Museum Göttingen (GMG), and five specimens were from the Senckenberg Museum Frankfurt (SMF).
18 snakefly larvae, preserved in twelve pieces of Cretaceous amber from Myanmar, were documented. Specimens are either from the Palaeo-Evo-Devo Research Group Collection of Arthropods, Ludwig-Maximilians-Universität München (PED) and were legally purchased on ebay.com from the trader burmite-miner. The remaining specimens come from the collection of one of the authors (Patrick Müller; BUB).
Images of additional extant and fossil specimens were provided by enthusiasts, hobby scientists, or collectors and retrieved from the literature. Details about these are indicated in the Results section. All specimens included in this study are listed in detail in Supplementary Table 1 and in Supplementary Text 1.
Documentation methods
The four extant specimens were documented on a Keyence BZ-9000 fluorescence microscope. Several adjacent image stacks were recorded for each specimen, which were subsequently fused with CombineZP and stitched with Photoshop Elements CS3 (composite imaging; see Haug et al. 2008, 2011; Kerp and Bomfleur 2011). Some specimens were documented two times in the same position, but with different exposure times to make all details visible. The resulting composite images were virtually overlain subsequently (Haug et al. 2013).
All fossil specimens, i.e. specimens in amber, directly investigated were documented on a Keyence VHX-6000 digital microscope. Each specimen was documented from each accessible direction with four settings: (1) with cross-polarised coaxial light in front of white background, (2) same illumination in front of black background, (3) with ring light in front of white background, (4) same illumination in front of black background (Haug and Haug 2019). Also here, several image stacks were recorded for each specimen, which were fused and stitched with the built-in software.
Approach
Up to eight dimensions of a specimen were measured on the images (Fig. 1). This includes length of the head capsule, length of the pronotum, length of the remaining trunk, maximum width of the head capsule, maximum width of the pronotum, maximum width of the remaining trunk, length of the antenna, length of the total body (excluding appendages).
Based on these values, ratios were calculated. While the use of ratios has certain shortcomings, the type and structure of available data prohibited the use of other, more sophisticated approaches (see next paragraph). Ratios were analysed with simple methods in OpenOffice 4.0.1 and with multivariate methods in PAST 4.03. We compared three different groups represented by snakefly larvae from the modern fauna, from the Eocene, and from the Cretaceous. Two different sub-sets of the dataset were analysed differently: sub-data-set 1 included all specimens of which all dimensions could be measured, sub-data-set 2 included all specimens of which head width, head length, and antennae length could be measured.
Calculating ratios, as done here, is generally thought to be an inferior approach for removing size effects from a sampled data. More sophisticated methods, such as the Burnaby-Back-Projection, are readily available (Burnaby 1966; Rohlf and Bookstein 1987; McCoy et al. 2006; Blankers et al. 2012; Eberle et al. 2014), yet require quite different or additional types of data. Most notably, absolute sizes would be needed to apply the Burnaby-Back-Projection and comparable methods, thereby reducing our data set considerably, as many specimens, particularly those from the literature, often lack such information. For the current material, ratios are the most available and reliable data that permit analysis without reducing the data set to a size in which it is no longer informative. Given this, we believe that the shortcomings of ratios are acceptable costs that need to be accepted in the current situation and serve as a sufficiently meaningful and reliable proxy for more refined metrics. We used Principal Component Analysis as well as exploring dimensions directly. The rather low number of input dimensions makes such analysis possible. Due to the incomplete nature of some specimens, we have two different types of sub-data-sets: one that includes all specimens for which measurement of all dimensions was possible, while the other included more specimens but with only three dimensions measured. Regardless, the overall resulting picture is quite similar.
Results
Structure of the two sub-data-sets
In total, we inspected 52 extant immatures (Figs. 2, 3, 4, 5); 49 were included into sub-data-sets 1 and 2. In addition there are 21 Eocene specimens (Figs. 6, 7, 8, 9, 10, 11, 12, 13, 14); 20 were included into sub-data-set 1, and 21 were included into sub-data-set 2. Of 32 Cretaceous specimens (Figs. 15, 16, 17, 18, 19, 20, 21, 22, 23, 24, 25, 26), 21 were included into sub-data-set 1, and 31 were included into sub-data-set 2.
Extant immatures of snakeflies, CeNak/LIB collection, Hamburg; all composite autofluorescence images. a Specimen 1, larva, ventral view. b–e Specimen 2, very late larva, pre-pupa. b Ventral view. c Dorsal view. d Close-up on head in ventral view. e Details of trunk appendages. 1–4 antenna elements 1–4, cx coxa, fe femur, hc head capsule, lb labium, md mandible, mp maxilla palp, ms mesothorax, mt metathorax, pt prothorax, ta tarsus, ti tibia, tr trochanter
Extant immatures, pupae, of snakeflies, CeNak/LIB collection, Hamburg; all composite autofluorescence images. a, b Ventral view. c, d Dorsal view. a, c Specimen 3, female. b, d Specimen 4, male. a3–9 abdomen (posterior trunk) segments 3–9, hc head capsule, ms mesothorax, mt metathorax, ov ovipositor, pt prothorax, w developing wing
Extant larvae of snakeflies, not to scale. a, c, d provided by Pierre Gros. a Specimen 42, Parainocellia bicolor. b Specimen 46, Xanthostigma xanthostigma, provided by Maria Justamond. c Specimen 43, Raphidia sp., d Specimen 44, Parainocellia bicolor. e Specimen 47, Raphidia sp., provided by Mark Leppin. f Specimen 48, Raphidia sp., provided by Nicholas Wiser. g Specimen 49, Xanthostigma xanthostigma, provided by Paul Kennedy
Snakefly larvae preserved in Baltic amber, not to scale. a, b Specimen 62, provided by Marius Veta (ambertreasure4u.com). c–f Specimens provided by Jonas Damzen (amberinclusions.eu). c Specimen 63. d Specimen 64. e Specimen 65. f Specimen 66. a1–9 abdomen (posterior trunk) segments 1–9, fe femur, hc head capsule, ms mesothorax, mt metathorax, pt prothorax, ta tarsus, te trunk end, ti tibia
Snakefly larva preserved in Baltic amber, GPIH_Scheele_1242_TK-NR_647, specimen 67. a Ventral view. b Colour-marked version of (a). c Dorsal view. d Close-up on anterior head region, ventral view. e Second trunk appendage. f Third trunk appendage. a2–8 abdomen (posterior trunk) segments 2–8, cx coxa, fe femur, hc head capsule, md mandible, mp maxilla palp, ms mesothorax, mt metathorax, pt prothorax, ta tarsus, te trunk end, ti tibia, tr trochanter
Snakefly larva preserved in Baltic amber, GMG 22866 collection, specimen 68. a Dorsal view. b Colour-marked version of (a). c Close-up on anterior head region, dorsal view. d Trunk appendages. 1–4 antenna elements 1–4, a1–9 abdomen (posterior trunk) segments 1–9, cx coxa, fe femur, hc head capsule, lr labrum, md mandible, mp maxilla palp, ms mesothorax, mt metathorax, pt prothorax, ta tarsus, te trunk end, ti tibia, tr trochanter
Snakefly larva preserved in Baltic amber, SMF 239, specimen 69. a Dorsal view. b Colour-marked version of (a). c First trunk appendage; arrow points to claws. d Second trunk appendage. e Third trunk appendage. a1–9 abdomen (posterior trunk) segments 1–9, at antenna, fe femur, hc head capsule, ms mesothorax, mt metathorax, pt prothorax, ta tarsus, te trunk end, ti tibia
Snakefly larva preserved in Baltic amber, SMF 2022, specimen 70. a Largely ventral view, posterior trunk folded anteriorly. b Colour-marked version of (a). c Close-up on anterior head region, ventral view. d Close-up on distal region of trunk appendage 1. 1–4 antenna elements 1–4, a2–8 abdomen (posterior trunk) segments 2–8, cx coxa, fe femur, hc head capsule, ms mesothorax, mt metathorax, pt prothorax, te trunk end, tr trochanter
Snakefly larva preserved in Baltic amber, SMF 2024, specimen 71. a Dorsal view. b Colour-marked version of (a). c Close-up on anterior trunk region, ventral view. d Close-up on distal region of antenna. a2–8 abdomen (posterior trunk) segments 2–8, cx coxa, fe femur, hc head capsule, ms mesothorax, mt metathorax, pt prothorax, ta tarsus, te trunk end, ti tibia, tr trochanter
Snakefly larva preserved in Baltic amber, SMF 10524, specimen 72. a Ventral view. b Colour-marked version of (a). c Second trunk appendage. d First right trunk appendage. e Antenna. f Third trunk appendage. g Close-up on head region, ventral view. h First left trunk appendage. Arrows in all images point to claws on appendages. at antenna, fe femur, hc head capsule, lp labium palp, md mandible, mp maxilla palp, ms mesothorax, mt metathorax, pt prothorax, ta tarsus, ti tibia, tr trochanter
Snakefly larva preserved in Baltic amber, SMF 10525, specimen 73. a Ventral view. b Dorsal view. c Colour-marked version of (b). d Close-up on first trunk appendage. e Close-up on second trunk appendage. f Close-up on anterior part of the head; arrows point to palps of labium. a1–9 abdomen (posterior trunk) segments 1–9, at antenna, hc head capsule, lp labium palp, ms mesothorax, mt metathorax, pt prothorax, te trunk end
Snakefly larvae preserved in Myanmar amber. a, b BUB 3069, specimen 87 (from Haug et al. 2020a). a Ventral view. b Colour-marked version of (a). c–f PED 0039, specimen 88. c Dorsal view. d Colour-marked version of (c). e Close-up on head. f Close-up on third trunk appendage. 1–5 antenna elements 1–5, a2–8 abdomen (posterior trunk) segments 2–8, fe femur, hc head capsule, md mandible, mp maxilla palp, ms mesothorax, mt metathorax, pt prothorax, ta tarsus, te trunk end, ti tibia, tr trochanter
Snakefly larva preserved in Myanmar amber, PED 0064, specimen 89. a Ventral(?) view. b Colour-marked version of (a). c Close-up on head. 2–5 antenna elements 2–5, a2–8 abdomen (posterior trunk) segments 2–8, hc head capsule, lr labrum, md mandible, mp maxilla palp, ms mesothorax, mt metathorax, pt prothorax, ta tarsus, te trunk end, ti tibia, tr trochanter
Snakefly larvae preserved in Myanmar amber. a PED 0105-I, specimen 90, in dorsal view; note that also PED 0105-II, specimen 91, is visible (see Fig. 18a). b Colour-marked version of (a). c Close-up on anterior part of head. 2–3 antenna elements 2–3, at antenna, fe femur, hc head capsule, lr labrum, md mandible, mp maxilla palp, pt prothorax
Snakefly larvae preserved in Myanmar amber. a, b PED 0105-II, specimen 91; note that also PED 0105-I, specimen 90, is visible (see Fig. 17a). a Dorsal view. b Colour-marked version of (a). c–e PED 0105-III, specimen 92. c Ventral view. d Colour-marked version of (c). e Close-up on anterior head region. 1–5 antenna elements 1–5, fe femur, hc head capsule, lb labium, lr labrum, md mandible, mp maxilla palp, pt prothorax, ti tibia
Snakefly larva preserved in Myanmar amber, BUB 3070, specimen 93. a Dorsal view. b Colour-marked version of (a). c Close-up on third trunk appendage. 1–5 antenna elements 1–5, fe femur, hc head capsule, lr labrum, md mandible, mp maxilla palp, ms mesothorax, mt metathorax, pt prothorax, ta tarsus, ti tibia, tr trochanter
Snakefly larvae preserved in Myanmar amber. a, b BUB 3129, specimen 94. a Dorsal view. b Colour-marked version of (a). c, d BUB 3145, specimen 95. c Dorsal view. d Colour-marked version of (c). 1–5 antenna elements 1–5, a2–8 abdomen (posterior trunk) segments 2–8, fe femur, hc head capsule, md mandible, mp maxilla palp, ms mesothorax, mt metathorax, pt prothorax, ta tarsus, te trunk end, ti tibia, tr trochanter
Snakefly larvae preserved in Myanmar amber. a–c BUB 3141; specimen 96. a Dorsal view. b Colour-marked version of (a). c Close-up on anterior head region. d, e BUB 3130; specimen 97. d Dorsal view. e Colour-marked version of (d). 1–4 antenna elements 1–4, a1–8 abdomen (posterior trunk) segments 1–8, fe femur, hc head capsule, lr labrum, md mandible, mp maxilla palp, ms mesothorax, mt metathorax, pt prothorax, ta tarsus, te trunk end, ti tibia, tr trochanter
Snakefly larva preserved in Myanmar amber; BUB 3131; specimen 98. a Dorsal view. b Colour-marked version of (a). c Close-up on second trunk appendage. d Close-up on pronotum; note the surrounding setae. 2–4 antenna elements 2–4, a2–8 abdomen (posterior trunk) segments 2–8, fe femur, hc head capsule, lr labrum, md mandible, mp maxilla palp, ms mesothorax, mt metathorax, pt prothorax, ta tarsus, te trunk end, ti tibia, tr trochanter
Snakefly larva preserved in Myanmar amber; BUB 3135; specimen 99. a Dorsal view. b Colour-marked version of (a). c Close-up on anterior head region in dorsal view. d Close-up on anterior head region in ventral view. e Close-up on second trunk appendage. f Close-up on third trunk appendage. 2–4 antenna elements 2–4, a1–9 abdomen (posterior trunk) segments 1–9, cx coxa, fe femur, hc head capsule, lr labrum, md mandible, mp maxilla palp, ms mesothorax, mt metathorax, pt prothorax, ta tarsus, te trunk end, ti tibia, tr trochanter
Snakefly larva preserved in Myanmar amber; BUB 3140; specimen 100. a Dorsal view. b Colour-marked version of (a). c Close-up on head. d Close-up on trunk appendages. 1–4 antenna elements 1–4, a2–8 abdomen (posterior trunk) segments 2–8, hc head capsule, md mandible, mp maxilla palp, ms mesothorax, mt metathorax, pt prothorax, ta tarsus, te trunk end, ti tibia
Snakefly larvae preserved in Myanmar amber. a, b BUB 3346-I; specimen 101. a Ventral view. b Colour-marked version of (a). c, d BUB 3346-II; specimen 102. c Ventral view. d Colour-marked version of (c). e Four (I–IV) of the five specimens in amber BUB 3346 visible here. a1–7 abdomen (posterior trunk) segments 1–7, hc head capsule, md mandible, ms mesothorax, mt metathorax, pt prothorax
Snakefly larvae preserved in Myanmar amber. a, b BUB 3346-IV; specimen 104. a Ventral view. b Colour-marked version of (a). c, d BUB 3346-III; specimen 103. c Latero-ventral view; note that also BUB 3346-I, specimen 101, is visible (see Fig. 25a). d Colour-marked version of (c). e, f BUB 3346-V; specimen 105. e Dorsal view. f Colour-marked version of €. 2–4 antenna elements 2–4, a2–6 abdomen (posterior trunk) segments 2–6, cx coxa, fe femur, hc head capsule, ms mesothorax, mt metathorax, p palp, pt prothorax
Differences through time, sub-data-set 1: simple analysis (Fig. 27 )
-
1.
Relative head length. The smallest range is found in the modern fauna, the largest range in the Cretaceous, entirely spanning the range of the modern forms. The Eocene fauna has a smaller range, lacking some of the shorter heads, but having also examples of longer heads, neither present in the modern fauna nor in the Cretaceous.
-
2.
Relative pronotum length. The modern fauna has a quite small range, yet in the Eocene it is even smaller and shifted towards longer pronotum lengths. The range of pronotum lengths in Cretaceous larvae is largest, covering the range of modern and Eocene larvae, but also including longer ones.
-
3.
Relative length of posterior trunk. The range of the modern larvae is moderate, that of the Eocene larvae is smaller and shifted towards shorter trunks, including some that are shorter than any of the modern forms. The range of the Cretaceous larvae is again the largest, covering the entire range of the modern forms and most of that of the Eocene larvae, excluding only the small area represented by the ones with the very short trunks.
-
4.
Relative width of head. The modern larvae occupy a quite small range concerning head width. The Eocene larvae hardly diverge from this range. Again the range represented by the Cretaceous larvae is the largest, covering the entire range of the other two groups.
-
5.
Relative width of the pronotum. This ratio shows the overall smallest differences between the three groups. The range is smallest for the modern larvae. The ranges of the Eocene and Cretaceous larvae are virtually identical, only slightly larger than the range of the modern larvae and covering it entirely.
-
6.
Relative width of trunk. The range of the modern larvae concerning trunk width is quite large. That of Eocene larvae is smaller, but includes more slender forms not present in the modern fauna. The range of the Cretaceous larvae is largest and covers the range of the modern and of the Eocene larvae.
-
7.
Relative antenna length. The range of relative antenna length of the modern larvae is again the smallest, including very short antennae not present in the Eocene and Cretaceous larvae. The range of the Eocene larvae is larger and is shifted towards longer antennae. This is even more expressed in the Cretaceous larvae where the range is significantly larger and even more shifted towards long antennae. In the Cretaceous, larvae with shorter antennae, as present in the Eocene and even more so in the modern fauna, are missing.
Overall, the range of Cretaceous larvae is larger than that of the modern-day larvae. In the first six ratios, the range of the modern larvae is fully covered by those of the Cretaceous larvae. Although this is not so simple and clear for the Eocene larvae, we indeed see a clear decline of variation in the first six ratios.
The variation of the relative length of antenna also decreases clearly from the Cretaceous to the Eocene and even more towards the modern fauna. Additionally, the range is progressively shifted towards shorter forms, hence showing not only a simple loss of forms, but also the appearance of new morphologies with quite short antennae.
To see whether certain ratios were depending on size, we plotted each ratio over absolute size for the sub-data set where absolute size was available (Supplementary Table 1). The regressions have very flat slopes (hl/bl ≈ 0.00050; pl/bl ≈ − 0.00027; rtl/bl ≈ − 0.00024; hw/bl ≈ 0.00056; pw/bl ≈ 0.00042; rtw/bl ≈ 0.00152; al/bl ≈ 0.00085) and very low R2-values (hl/bl ≈ 0.018; pl/bl ≈ 0.005; rtl/bl ≈ 0.002; hw/bl ≈ 0.025; pw/bl ≈ 0.021; rtw/bl ≈ 0.069; al/bl ≈ 0.005), indicating that there is little to no size dependence.
Differences through time, sub-data-set 1: multivariate analysis
The principal component analysis (PCA) provides seven principal components (PCs; Fig. 28, Supplementary Table 2, Supplementary Fig. 1).
PC1 explains 52.8% of the entire variation. It is dominated by the relative length of the antenna (0.985). The modern fauna has a rather small range of PC1. The Eocene fauna has a similarly small range, but is slightly shifted upwards compared to the modern fauna. The Cretaceous fauna has a much larger range than the other two, about three times as large, reaching far further up than the range of the other two faunas. The lower values of PC1 in modern larvae are not covered by the other two faunas, and the lower values of the Eocene are not covered by the Cretaceous fauna. This result strongly resembles the result of the simple analysis of the relative antenna length (compare with Fig. 27, lower right).
PC2 explains 26.4% of the entire variation. It is dominated by the relative head length (0.450), but additionally strongly influenced to about equal amounts by relative length of pronotum (0.270), relative width of the head (0.254), relative width of the pronotum (0.257), and relative width of the remaining trunk (0.243). The range of PC2 of modern larvae is larger than that of the Eocene larvae, especially in the negative values. For Cretaceous larvae, the range is the largest, almost covering the ranges of both modern and Eocene larvae, but with a larger range in the negative values.
PC3 explains 12.0% of the entire variation. It is strongly dominated by the relative width of the remaining trunk (0.860). The range of PC3 for the modern larvae is rather small. The range of the Eocene larvae is slightly larger, but especially shifted to the more negative values. In Cretaceous larvae, the range is larger than in the other two faunas, covering the ranges of both and extending further into the positive values.
PC4 explains 4.4% of the entire variation. It is mostly influenced by the relative width of the head (0.673) and relative width of the pronotum (0.447). The range of PC4 for modern larvae is very small. The range of Eocene larvae is a bit larger, extending further into positive and negative values. For Cretaceous larvae, the range is still larger, also extending further into both directions.
PC5 explains 3.6% of the entire variation. It is mostly dominated by the relative length of the pronotum (0.618) and by the relative width of the pronotum (0.357). The range of PC5 is rather similar for all larvae, but smallest in the modern larvae and largest in the Cretaceous larvae.
PC6 explains 0.8% of the entire variation. It is dominated by the width of the pronotum (0.768). The range of PC6 is relatively small for extant larvae and almost the same, but slightly smaller for Eocene larvae. The range of Cretaceous larvae is slightly larger than for the two other faunas, especially reaching further into the negative values, and covering the ranges of both.
PC7 explains 1.26*10–27 of the entire variation. It is equally dominated by the relative lengths of head, pronotum, and the remaining trunk (each about 0.577). The range of PC7 is very small and similar for all faunas.
When PC2 is plotted over PC1, extant and Eocene larvae plot in about the same area (Fig. 29). Larvae of both faunas plot almost exclusively in the negative PC1 values, extant larvae occupy a slightly larger range in the negative PC2 values. The Cretaceous larvae occupy a rather similar PC2 range, but a much larger PC1 range, almost exclusively in the positive PC1 values. Two Cretaceous larvae, specimens 75 (Engel 2002; Fig. 29a) and 87 (BUB 3069; Haug et al. 2020a; Fig. 29b), that appeared as possessing an extreme morphology in earlier studies, plot in different areas here. Specimen 75 plots rather centrally among the Cretaceous larvae, while specimen 87 plot much further to the right. Two other specimens, specimen 89 (PED 0064; Fig. 29c) and 97 (BUB 3130; Fig. 29d), plot in more extreme positions due to their very long antennae and additionally an almost square-shaped head in specimen 89.
Scatter plot of the PC2 values over PC1 values of the principal component analysis of sub-data-set 1. The highlighted specimens have been recognised as morphologically extreme already in earlier studies (a, b) or in this study (c, d). a Specimen 75 (modified from Engel 2002). b Specimen 87 (BUB 3069; modified from Haug et al. 2020a). c Specimen 89 (PED 0064). d Specimen 97 (BUB 3130)
Differences through time, sub-data-set 2
As not all values could be measured on all specimens, for sub-data-set 2 only head width, head length, and antennae length were included; this allowed to include more incompletely preserved fossil specimens. Note that antennae length and head length (and partly also head width) were dominating values in the analyses of sub-data-set 1.
When plotting the ratio of antennae length/head length over the ratio of head width/head length, extant and Eocene larvae plot in almost the same area with small relative antennae lengths (Fig. 30). The Eocene larvae occupy a slightly larger area at the smaller relative head widths. Some Cretaceous larvae also plot in the same area as the extant and Eocene larvae, but most of them plot further up, at larger relative antennae lengths, and partly also at relatively broader and in few cases slimmer heads.
Scatter plot of the ratio of antennae length/head length over the ratio of head width/head length of sub-data-set 2. The highlighted specimens are the same as those highlighted in Fig. 29. a Specimen 75 (modified from Engel 2002). b Specimen 87 (BUB 3069; modified from Haug et al. 2020a). c Specimen 89 (PED 0064). d Specimen 97 (BUB 3130)
All four specimens mentioned explicitly above in the multivariate analysis plot here in extreme positions. Specimen 75 (Engel 2002; Fig. 30a) plots at a rather small relative head width, specimen 87 (BUB 3069; Haug et al. 2020a; Fig. 30b) plots at a relative large antennae length. Specimen 89 (PED 0064; Fig. 30c) and 97 (BUB 3130; Fig. 30d) plot at still further extreme positions due to the very long antennae in specimen 89 and the rather long antennae and very broad head in specimen 97.
Discussion
How uniform are snakefly larvae?
Extant snakefly larvae are largely rather uniform, and many fossil forms greatly resemble such modern-day forms. Nonetheless, some prior observations reported Cretaceous larvae that deviated at least in certain aspects from this seemingly stereotypical morphology. Specimen 75 described by Engel (2002) is certainly unusual compared to modern forms, possessing a rather slender, elongate head not seen in modern larvae, and quite prominent antennae, while in modern forms, the antennae are usually rather short. Another specimen from Burmese amber like specimen 75 is specimen 87, reported by Haug et al. (2020a), which possesses even more prominent and larger antennae. Haug et al. (2020a) established various metrics for characterizing the exceptional nature of these two individuals, more conclusively demonstrating that such Cretaceous morphologies lacked analogs among modern snakeflies.
Our significantly expanded data set further emphasizes the notion that during the Cretaceous snakefly larvae occupied a greatly expanded range of morphospace. These Cretaceous taxa exhibited a range of morphological diversity (≈ disparity) that has subsequently contracted, likely in connection with the loss of species diversity and the extinction of unique forms, perhaps also linked to biologies and life histories no longer represented among raphidiopteran diversity today. Interestingly, although the aforementioned specimens 75 and 87 are here further demonstrated to be clearly set apart from modern-day forms (Engel 2002; Haug et al. 2020a), they are only a subset of an even wider morphological variety. Among the new larvae reported there are those that are even more exceptional, significantly expanding the overall morphospace occupied (Figs. 29c, d, 30c, d).
Changes over time
Our results demonstrate that larval forms in the Cretaceous exhibit a much larger morphological diversity (≈ disparity) relative to more modern forms. While this is most apparent in the length of the antennae and the head shape, it is also apparent in other factors. However, some of the variation may be explained by different preservational aspects of the thorax and certainly the abdomen in several of the fossil larvae. Nonetheless, the analysis restricted to the more heavily sclerotised elements, i.e., head capsule and antennae, provides a rather similar perspective.
Specimens from the Eocene show a much more restricted morphospace, while remaining slightly greater than that of modern larvae. This is especially interesting as the sub-data-set for both fossil groups is significantly smaller than the sub-data-set of the modern forms, suggesting that sample-size corrections are not necessary (refer to Haug et al. 2020b).
It is apparent that over evolutionary time the overall body shape of snakefly larvae did not change significantly, i.e., these larvae seem to have always been quite slender. By contrast, the variability in relative proportions of the head shape and antennae have changed significantly during the last 100 million years. Indeed, the considerable morphological diversity observed during the Cretaceous decreased significantly by at least the Eocene and then continued to retract through to the present day. It is possible that this is reflective of differing sensory requirements and different modes of predation among these larvae. Certainly, some developmental requirements changed considerably during this expanse of time as all of the known fossil snakefly larvae occur in palaeo-climates inconsistent with the necessary exposure to extended freezing to bring on pupation that is otherwise so characteristic of our modern fauna (Gruppe et al. 2020). Accordingly, the expectation that there were similar or even related changes in diet, predation specialization and behaviours, and ecology seems well founded. In fact, the loss of diversity was perhaps brought about by the loss of species as former groups, such as Baissopteridae and Mesoraphidiidae, were eventually replaced by a restricted group among this diversity, namely the group Neoraphidioptera (Liu et al. 2014; Engel et al. 2018). This restriction would have been both in terms of phylogenetic diversity as well as morphological diversity, and perhaps also in ecology, behaviour, and physiology.
Evolutionary mechanisms
Evolution acts via subtle changes and processes. Heterochrony describes a set of slight evolutionary changes to developmental timing. We might therefore assume that heterochrony might have played a role in forming the morphology of snakefly larvae. Haug et al. (2020a) suggested that the large antennae in specimen 87 might represent a specialisation and that small antennae (as seen in modern-day larvae) might be ancestral, but the significantly larger data set available with this study suggests otherwise. In fact, comparing the ratios for relative antennal length among Cretaceous, Eocene, and extant larvae indicates a clear trend to less variability, but also progressively shorter antennae. This suggests that ancestrally the antennae of snakefly larvae were longer and that the shorter antennae seen in modern larvae is the derived condition, at least relative to the raphidiomorphans. Naturally, this implies that the phylogenetic distribution for this trait is that a number of early consecutive-diverging snakefly lineages had longer larval antennae relative to more derived and younger monophyletic groups. Unfortunately, we do not know the phylogenetic relationships among the fossils included in our study, not even allowing identification to higher groups (e.g., family). Moreover, larvae remain unknown for the diversity of Jurassic species, and particularly those of the extinct group Priscaenigmatomorpha (Engel 2002), and these could alter our conclusions once such material is discovered and put into a proper phylogenetic context with the present material. Nonetheless, even in the absence of a phylogeny based on larval characters, the general distribution of antennal lengths and their changes through time remain consistent with the pattern and character polarity outlined herein.
Naturally, shorter appendages can be interpreted as paedomorphic, i.e., retained earlier developmental morphologies in later stadia. This may be achieved by the later onset of development (post-displacement), a shorter developmental time (progenesis), or simply slower development (neoteny) of the antennae (Webster and Zelditch 2005). With the available data, we cannot differentiate between these possibilities, and reconstructed larval sequences would be necessary for making such a differentiation. For the moment, it seems likely that some of the fossil larvae may represent different stages of a single species (e.g., Figs. 16 and 21d, e), and therefore there should be the potential for exploring such an analysis in the future. In particular, several specimens trapped in a single piece of amber should have a greater likelihood of being conspecific (e.g., Figs. 18, 19, 25, 26). In addition, fossil larvae with a more extreme morphology may be good candidates for identifying conspecifics in other pieces (e.g., Figs. 16 and 21d, e). For example, it is tantalizing to consider those larvae with elongate heads (e.g., specimen 75) with species such as Rhynchobaissoptera hui (Lu et al. 2020), although as we note below there is some degree of disconnect in certain lineages between larval head shape and that of the corresponding adult.
It would be fascinating and perhaps revealing to expand the comparison to include pupae and adults. It may be possible that this type of heterochronic shift of the antenna only affects larval stages, while the morphology of the adult remains unchanged (cf. Haug et al. 2016; Haug 2020a, b). Modern-day pupae have clearly longer antennae than modern-day larvae, but the antennae are differently structured, with many small antennomeres, while the long antennae of the fossil larvae have only a few but often long antennomeres.
Likewise, the varying head shapes of the fossil larvae would be interesting to set in a framework including adults. Modern pupae apparently have already a longer head and a relatively shorter thorax (the latter is also observed in the retraction of the thorax when forming the pre-pupa; Fig. 2b, c; see also Haug et al. 2020c for a comparable process in lacewings). It could follow then that the higher variability in fossil larvae is the result of differential timing of the point from which the elongation of the head capsule begins. Alternatively, it is possible that in the past snakefly adults had a similarly greater head-shape variability, a matter which should be addressed in future analyses.
It has not escaped our notice that head shape, where we observe so much variation and changes in larvae, is correlated in adults with differences between the two major modern lineages (families) of Raphidioptera (Neoraphidioptera). Among the traits that differentiate the two lineages is an overall difference in the shape of the head capsule posteriorly: those of Inocellidae are rather robust and broad posteriorly, with broadly rounded posterolateral angles, while those of Raphidiidae taper more gently caudally. In the Eocene fauna, there is at least one group that intermingles features of these two groups in terms of such head shape, specifically the group Electrinocelliinae (Engel 1995). Despite these differences, the head capsules of larvae of these two groups are more similar, and all are quite robust and broad posteriorly, at least relative to that observed in adults of Raphidiidae. This may be an indication that the broader head morphology (in both adults and larvae) is plesiomorphic, at least for this group, with that observed in Raphidiidae representing a specialized novel character state. Alternatively, there may be some degree of decoupling between larval and adult head morphology; perhaps not surprising given the dramatic developmental rearrangement between the larval and pupal/adult stadia. Nonetheless, such matters could be more fully explored in detail by the potential future expanded analyses outlined here.
It would also be beneficial to discover fossil snakefly pupae for expanding the comparisons initiated herein. As noted, snakefly pupae are quite active with considerable shared resemblance to the adult. In many other holometabolan lineages, the pupa is quiescent and differs more starkly from the adult (e.g., Beutel et al. 2014; Saltin et al. 2016). It remains unclear whether these two aspects are ancestral or derived for the broader group. Fossil pupae could provide an important insight into this aspect of neuropteridan, and perhaps greater holometabolan, evolution and would certainly allow us to enrich our comparisons.
While our data are still limited for answering such evolutionary or palaeo-evo-devo questions and despite the difficulties of placing fossil larvae in robust phylogenetic estimates (but see Badano et al. 2018), we can already extract some signal indicating paedomorphosis in the evolution of modern-day snakefly larvae.
Snakefly diversity
It seems generally accepted that snakeflies were more diverse in the past (Engel 1995: 187; Aspöck 2002: 35, 36; Aspöck and Aspöck 2007: 478–480, 2009: 53; Liu et al. 2016; Engel et al. 2018). The taxonomic view on this aspect is difficult. In few cases does pure species counting as a basis for taxonomic comparison between time periods provide a clear view. The group Dinosauria is a notable example. While most people believe their maximal diversity ended at the Cretaceous-Paleogene transition, the more than 10,000 extant bird species clearly outnumber formally described fossil species by a factor of 10, suggesting that dinosaurian diversity (as represented by their ingroup Aves) experienced its greatest diversification during the Cenozoic! Only a few lineages prominently show a large number of fossil species against a significantly smaller extant number (notable examples among insects include Mastotermitidae, Megalyridae, Scolebythidae: Grimaldi and Engel 2005). Given that there are about 248 extant and only 100 extinct species of Raphidioptera (Liu et al. 2016; Engel et al. 2018; Lu et al. 2020), the raw number of species does not alone support the conclusion of declining diversity. Furthermore, the documentation of declining diversity is not a mere comparison of the modern fauna versus the cumulative total of all species that existed prior to the present (although most often researchers rely solely on a lumping of all past faunas). Instead, it is more properly an assertion that in progressively younger faunas each has fewer and fewer species, or at least an overall trend in such a direction. From this perspective, the picture is even murkier as all of the known fossil faunas for Raphidioptera are a mere fraction of the modern diversity, and there are no sufficient data to establish such a trend.
Accordingly, where is the taxonomic signal for a higher diversity in the past? In recent history, neuropterists have rightly considered the number of extinct species described to be a mere fraction of the diversity during any given moment in the past and therefore concluded that, when lumping all palaeofaunas into one, the 100 or so fossil species are reflective of a far greater total diversity. Under such a scenario, if the 100 represented even a tenth of the actual fauna over that period, then certainly the numbers dwarf that of the modern fauna. Nonetheless, data are insufficient to apply such an extrapolation to any given palaeofauna as the amount of material is scant and conclusions on high diversity in any one fauna become increasingly speculative. Thus, aside from such gross estimations, higher ranks have been used as rough proxies for diversity. Extant snakeflies have been classified into two higher groups, traditionally recognized as families, the fossil diversity into six, and this gives an impression of more diversity (Engel 1995, 2002; Liu et al. 2016). Naturally, higher groups are to some degree subjective and reflective of the degree to which the individual taxonomist circumscribes the observed diversity. Two independent taxonomists recognizing the same species and monophyletic groups could arrive at radically different numbers of higher taxa solely based on how broadly or narrowly each circumscribed the groups. Each opinion is, ideally, founded in valid morphological observations among the living and fossil diversity, and ideally organized within a phylogenetic framework. Nonetheless, the raw numbers are somewhat artificial. For example, if each of the groups now ranked as subfamilies of Neoraphidioptera was elevated to familial rank (resulting in Electrinocelliidae, Succinoraphidiidae, Raphidiidae, and Inocelliidae), then right away the apparent change in diversity has diminished when comparing the Cenozoic and Mesozoic raphidiopteran faunas, and yet the morphological and phylogenetic observations remain unchanged. This would become even more dramatic if in-groups in Raphidiidae and Inocelliidae were similarly accorded such a rank. If a higher group would be identified as paraphyletic, then the validity of the distinction would only be further eroded in either direction in terms of changing diversity. Ranks are a tool for orientation in a greater phylogenetic outline and serve only as the most coarse and imprecise of qualitative proxies for diversity.
The approach presented here provides an alternative proxy, and although it has its own conceptual limitations, it at least offers some quantitative framework in which to observe changes in the absence of absolute (or even raw) species numbers from each time period. If morphological diversity is a proxy for species diversity in Raphidioptera, then the trend quantified herein is consistent with the traditional hypothesis of increased past diversity and that modern snakeflies are relict. Of course, morphological diversity outlining a large morphospace may be achieved by a few isolated and extremely divergent species rather than a greater number and variety (diversity) of species. Nonetheless, it is a more quantifiable and direct proxy for diversity than categorial ranks.
Our analysis permits us a view into larval diversity. Naturally, this poses some challenges as fossil larvae most often cannot be reliably identified to species or associated with species previously circumscribed on the basis of adults (but see a rare case in Batelka et al. 2021). Yet, in many holometabolans, the larvae represent the longest portion of the life cycle and therefore by some measures the most critical for avoiding harm and obtaining sustenance. Therefore the approach presented here may support earlier, taxonomy-based findings, but perhaps provides a more reliable perspective concerning the overall ecological role of the animals involved.
Quite intuitively, for a group assumed to have declining diversity, we found a trend toward declining morphological disparity. That is the opposite of that observed for ants where Cretaceous ants were less diverse and followed by significant diversification, and correspondingly exhibit a narrower morphospace relative to their more diverse modern counterparts (Barden and Grimaldi 2016). Nonetheless, in ants the Cretaceous morphospace overlaps considerably with modern ant morphospace (occupying about 10% of modern ant space). In the present case of snakefly larvae, the extant morphospace (PC1 and PC2) is only 23% of the size of the Cretaceous morphospace. The overlap is even smaller, only 7% of the Cretaceous morphospace is shared with modern snakefly larvae morphospace. Thus, in ants as diversity increased, more and more morphospace was explored, while in snakeflies, a once-broad swath of Cretaceous morphospace was eroded away as specific groups or specialized niches occupying portions of snakefly morphospace became extinct.
A broader view on Neuropterida
The snakeflies are sister to the monophyletic group Eidoneuroptera (= Megaloptera + Neuroptera) (Engel et al. 2018; Winterton et al. 2018). Megaloptera comprises the dobsonflies, fishflies, and alderflies, the larval forms of which are all aquatic predators. The fossil record of these larvae is quite scarce (Baranov et al. 2022), not easily facilitating a comparison of larval diversity or disparity. Neuroptera includes many different types of lacewings, and lacewing larvae are better represented in the fossil record (Pérez-de la Fuente et al. 2020). From a qualitative perspective, it is quite clear that in the past, there were many larval types of lacewings that are no longer present today (Pérez-de la Fuente et al. 2012b, 2016; Wang et al. 2016; Liu et al. 2016, 2018; Badano et al. 2018; Haug et al. 2019a, b, c), but at the same time, many quite modern forms also occurred (e.g., Wang et al. 2016; Haug et al. 2018; Pérez-de la Fuente et al. 2020), collectively providing for a larger overall diversity (including morphological diversity; see discussion in Haug et al. 2019b). Quantitative aspects also demonstrated that some larvae were already quite modern (Herrera-Flórez et al. 2020), while others have a morphology unparalleled in the modern fauna (Haug et al. 2019b, 2020b, c, d). Applying morphometric approaches (although based on outlines instead of measurements) revealed that in two lineages of Neuroptera, Psychopsidae (silky lacewings) and Nymphidae (split-footed lacewings), the larval diversity decreased significantly after the Cretaceous (Haug et al. 2020b, 2022). For long-necked antlions, i.e., the larvae of Crocinae (thread-winged lacewings), it remains partly unclear owing to challenges with preservation whether the diversity actually decreased; nonetheless, we also see a loss of certain larval morphologies (Haug et al. 2021).
The data obtained here for snakefly larvae reveal a similar trend, specifically that diversity of larval forms was significantly greater 100 million years ago. The loss of morphological diversity also indicates a loss of ecological diversity or ecological functions. As (almost) all modern larvae of Neuropterida (Raphidioptera, Megaloptera, and Neuroptera) are predators, we can conclude that the same was true for their many fossil species. Such a conclusion is well founded given that many of the predatory tools used by modern larvae are present in the fossil forms (e.g., scythe-like mandibles, piercing mandibles, etc.). A loss of diversity would mean a loss of predators, and while many were certainly generalist predators like many modern species, others were assuredly specialised for a particular type of prey, as is also observed in their modern neuropteridan counterparts. Thus, some aspects of the overall loss might reflect co-extinction, whereby specific types of preys went extinct and resulted in the loss of their specialist predators. For the moment, it remains unknown what preys were victimized by fossil snakeflies, but these could have included animals of the groups Acari (mites), Auchenorrhyncha (e.g., treehoppers, leafhoppers), Sternorrhyncha (e.g., whiteflies, greenflies, scale insects), and other preys often taken by modern snakeflies and certainly well represented in many of the palaeofaunas from which our snakefly material originated.
It has been asserted that Neuropterida is an ancient and relict group of Holometabola. Their larvae appear to attest to such an evolutionary pattern, at least during the last 100 million years.
Availability of data and material
All data are provided in the text, the figures, and the supplementary material.
Code availability
Not applicable.
References
Aspöck, H. 2002. The biology of Raphidioptera: A review of present knowledge. Acta Zoologica Academiae Scientiarum Hungaricae 48 (Suppl. 2): 35–50.
Aspöck, U., and H. Aspöck. 1999. Kamelhälse, Schlammfliegen, Ameisenlöwen. Wer sind sie? (Insecta: Neuropterida: Raphidioptera, Megaloptera, Neuroptera). Stapfia 60: 1–34.
Aspöck, U., and H. Aspöck. 2007. Verbliebene Vielfalt vergangener Blüte. Zur Evolution, Phylogenie und Biodiversität der Neuropterida (Insecta: Endopterygota). Denisia 20: 451–516.
Aspöck, H., and U. Aspöck. 2009. Raphidioptera—Kamelhalsfliegen. Ein Überblick zum Einstieg. Entomologica Austriaca 16: 53–72.
Aspöck, H., U. Aspöck, and H. Rausch. 1974. Bestimmungsschlüssel der Larven der Raphidiopteren Mitteleuropas (Insecta, Neuropteroidea). Zeitschrift für angewandte Zoologie [=Journal of Applied Zoologie] 61: 45–62.
Aspöck, H., U. Aspöck, and H. Rausch. 1975. Raphidiopteren-Larven als Bodenbewohner (Insecta, Neuropteroidea) (mit Beschreibungen der Larven von Ornatoraphidia, Parvoraphidia und Superboraphidia). Zeitschrift für angewandte Zoologie [=Journal of Applied Zoologie] 62: 361–375.
Aspöck, H., U. Aspöck, and H. Hölzel. 1980. Die Neuropteren Europas, vol. 2. Krefeld: Goecke and Evers.
Aspöck, H., X.-Y. Liu, and U. Aspöck. 2012. The family of Inocelliidae (Neuropterida: Raphidioptera). A review of present knowledge. Mitteilungen der Deutschen Gesellschaft für allgemeine und angewandte Entomologie 18: 565–573.
Ax, P. 1999. Das System der Metazoa, vol. 2. München: Urban and Fischer.
Badano, D., M.S. Engel, A. Basso, B. Wang, and P. Cerretti. 2018. Diverse Cretaceous larvae reveal the evolutionary and behavioural history of antlions and lacewings. Nature Communications 9: 3257. https://doi.org/10.1038/s41467-018-05484-y.
Baranov, V., C. Haug, M. Fowler, U. Kaulfuss, P. Müller, and J.T. Haug. 2022. Summary of the fossil record of megalopteran and megalopteran-like larvae, with a report of new specimens. Bulletin of Geosciences 97 (1).
Barden, P., and D.A. Grimaldi. 2016. Adaptive radiation in socially advanced stem-group ants from the Cretaceous. Current Biology 26 (4): 515–521. https://doi.org/10.1016/j.cub.2015.12.060.
Batelka, J., M.S. Engel, and J. Prokop. 2021. The complete life cycle of a Cretaceous beetle parasitoid. Current Biology 31: R101–R119. https://doi.org/10.1016/j.cub.2020.12.007.
Berendt, G.C. 1856. Die im Bernstein befindlichen organischen Reste der Vorwelt. Berlin: Nicolaische Buchhandlung.
Beutel, R.G., F. Friedrich, X.-K. Yang, and S.-Q. Ge. 2014. Insect Morphology and Phylogeny. Berlin and Boston: De Gruyter. https://doi.org/10.1515/9783110264043.
Blankers, T., D.C. Adams, and J.J. Wiens. 2012. Ecological radiation with limited morphological diversification in salamanders. Journal of Evolutionary Biology 25: 636–646. https://doi.org/10.1111/j.1420-9101.2012.02458.x.
Brohmer, P., P. Ehrmann, and G. Ulmer. 1930. Die Tierwelt Mitteleuropas. Insekten. i. Teil. IV. Band, 2. Lief. Steinfliegen, Uferfliegen, Geradflügler. Flechtlinge, Haarlinge, Fransenflügler. Leipzig: Quelle and Meyer.
Burnaby, T.P. 1966. Growth-invariant discriminant functions and generalized distances. Biometrics 22 (1): 96–110. https://doi.org/10.2307/2528217.
Dettner, K., and W. Peters. 2010. Lehrbuch der Entomologie, Teil 2. Heidelberg: Spektrum Akademischer Verlag.
Eberle, J., M. Renier, and D. Ahrens. 2014. The evolution of morphospace in phytophagous scarab chafers: No competition—no divergence? PLoS ONE 9 (5): e98536. https://doi.org/10.1371/journal.pone.0098536.
Eidmann, H., and F. Kühlhorn. 1971. Lehrbuch der Entomologie, 2nd ed. Berlin: Parey.
Engel, M.S. 1995. A new fossil snake-fly species from Baltic amber (Raphidioptera: Inocelliidae). Psyche 102: 187–193. https://doi.org/10.1155/1995/23626.
Engel, M.S. 2002. The smallest snakefly (Raphidioptera: Mesoraphidiidae): A new species in Cretaceous amber from Myanmar, with a catalog of fossil snakeflies. American Museum Novitates 3363: 1–22. https://doi.org/10.1206/0003-0082(2002)363%3c0001:tssrma%3e2.0.co;2.
Engel, M.S., S.L. Winterton, and L.C.V. Breitkreuz. 2018. Phylogeny and evolution of Neuropterida: Where have wings of lace taken us? Annual Review of Entomology 63: 531–551. https://doi.org/10.1146/annurev-ento-020117-043127.
Gepp, J. 1984. Erforschungsstand der Neuropteren-Larven der Erde (mit einem Schlüssel zur Larvaldiagnose der Familien, einer Übersicht von 340 beschreibenen Larven und 600 Literaturzitaten) In Progress in World's Neuropterology. Proceedings of the 1st International Symposium on Neuropterology (22–26 September 1980, Graz, Austria), eds. J. Gepp, H. Aspöck, and H. Hölzel, 183–239. Graz: privately printed.
Grimaldi, D. 2000. A diverse fauna of Neuropterodea in amber from the Cretaceous of New Jersey. In Studies on Fossils in Amber, with Particular Reference to the Cretaceous of New Jersey, ed. D. Grimaldi, 259–303. Leiden: Backhuys Publishers.
Grimaldi, D.A., and M.S. Engel. 2005. Evolution of the Insects. Cambridge: Cambridge University Press.
Gröhn, C. 2015. Einschlüsse im baltischen Bernstein. Kiel and Hamburg: Wachholtz.
Gruppe, A., and V. Abbt. 2018. Larval biology of Mongoloraphidia sororcula (H. Aspöck and U. Aspöck, 1966) (Neuropterida, Raphidioptera, Raphidiidae). Spixiana 41: 27–32.
Gruppe, A., V. Abbt, H. Aspöck, and U. Aspöck. 2020. Chilling temperatures trigger pupation in Raphidioptera: Raphidia mediterranea as a model for insect development. Spixiana 43: 119–126.
Haug, J.T. 2020a. Metamorphosis in crustaceans. In Developmental Biology and Larval Ecology. The Natural History of the Crustacea, vol. 7, ed. K. Anger, S. Harzsch, and M. Thiel, 254–283. Oxford: Oxford University Press.
Haug, J.T. 2020b. Why the term “larva” is ambiguous, or what makes a larva? Acta Zoologica 101: 167–188. https://doi.org/10.1111/azo.12283.
Haug, J.T., and C. Haug. 2019. Beetle larvae with unusually large terminal ends and a fossil that beats them all (Scraptiidae, Coleoptera). PeerJ 7: e7871. https://doi.org/10.7717/peerj.7871.
Haug, J.T., C. Haug, and M. Ehrlich. 2008. First fossil stomatopod larva (Arthropoda: Crustacea) and a new way of documenting Solnhofen fossils (Upper Jurassic, Southern Germany). Palaeodiversity 1: 103–109.
Haug, J.T., C. Haug, V. Kutschera, G. Mayer, A. Maas, S. Liebau, C. Castellani, U. Wolfram, E.N.K. Clarkson, and D. Waloszek. 2011. Autofluorescence imaging, an excellent tool for comparative morphology. Journal of Microscopy 244: 259–272. https://doi.org/10.1111/j.1365-2818.2011.03534.x.
Haug, C., K.R. Shannon, T. Nyborg, and F.J. Vega. 2013. Isolated mantis shrimp dactyli from the Pliocene of North Carolina and their bearing on the history of Stomatopoda. Bolétin de la Sociedad Geológica Mexicana 65: 273–284. https://doi.org/10.18268/BSGM2013v65n2a9 .
Haug, J.T., C. Haug, and R. Garwood. 2016. Evolution of insect wings and development—new details from Palaeozoic nymphs. Biological Reviews 91: 53–69. https://doi.org/10.1111/brv.12159.
Haug, J.T., P. Müller, and C. Haug. 2018. The ride of the parasite: A 100-million-year old mantis lacewing larva captured while mounting its spider host. Zoological Letters 4: 31. https://doi.org/10.1186/s40851-018-0116-9.
Haug, C., A.F. Herrera-Flórez, P. Müller, and J.T. Haug. 2019a. Cretaceous chimera–an unusual 100-million-year old neuropteran larva from the “experimental phase” of insect evolution. Palaeodiversity 12: 1–11. https://doi.org/10.18476/pale.v12.a1.
Haug, J.T., P. Müller, and C. Haug. 2019b. A 100-million-year old predator: A fossil neuropteran larva with unusually elongated mouthparts. Zoological Letters 5: 29. https://doi.org/10.1186/s40851-019-0144-0.
Haug, J.T., P. Müller, and C. Haug. 2019c. A 100-million-year old slim insectan predator with massive venom-injecting stylets—a new type of neuropteran larva from Burmese amber. Bulletin of Geosciences 94: 431–440. https://doi.org/10.3140/bull.geosci.1753.
Haug, J.T., P. Müller, and C. Haug. 2020a. A 100 million-year-old snake-fly larva with an unusually large antenna. Bulletin of Geosciences 95: 167–177. https://doi.org/10.3140/bull.geosci.1757.
Haug, G.T., C. Haug, P.G. Pazinato, F. Braig, V. Perrichot, C. Gröhn, P. Müller, and J.T. Haug. 2020b. The decline of silky lacewings and morphological diversity of long-nosed antlion larvae through time. Palaeontologia Electronica 23 (2): 39. https://doi.org/10.26879/1029.
Haug, J.T., V. Baranov, M. Schädel, P. Müller, P. Gröhn, and C. Haug. 2020c. Challenges for understanding lacewings: How to deal with the incomplete data from extant and fossil larvae of Nevrorthidae? (Neuroptera). Fragmenta Entomologica 52: 137–167. https://doi.org/10.4081/fe.2020.472.
Haug, J.T., P.G. Pazinato, G.T. Haug, and C. Haug. 2020d. Yet another unusual new type of lacewing larva preserved in 100-million-year old amber from Myanmar. Rivista Italiana Di Paleontologia e Stratigrafia 126: 821–832. https://doi.org/10.13130/2039-4942/14439.
Haug, G.T., V. Baranov, G. Wizen, P.G. Pazinato, P. Müller, C. Haug, and J.T. Haug. 2021. The morphological diversity of long-necked lacewing larvae (Neuroptera: Myrmeleontiformia). Bulletin of Geosciences 96: 431–457. https://doi.org/10.3140/bull.geosci.1807.
Haug, G.T., C. Haug, S. van der Wal, P. Müller, and J.T. Haug. 2022. Split-footed lacewings declined over time: indications from the morphological diversity of their antlion-like larvae. PalZ. Paläontologische Zeitschrift. https://doi.org/10.1007/s12542-021-00550-1.
Herrera-Flórez, A.F., F. Braig, C. Haug, C. Neumann, J. Wunderlich, M.K. Hörnig, and J.T. Haug. 2020. Identifying the oldest larva of a myrmeleontiformian lacewing—a morphometric approach. Acta Palaeontologica Polonica 65: 235–250. https://doi.org/10.4202/app.00662.2019.
Jacobs, W. 1998. Biologie und Ökologie der Insekten: ein Taschenlexikon / begr. von W. Jacobs, and M. Renner, 3. Aufl. überarb. von Honomichl, K. Stuttgart, Jena, Lübeck: Fischer.
Kerp, H., and B. Bomfleur. 2011. Photography of plant fossils—new techniques, old tricks. Review of Palaeobotany and Palynology 166: 117–151. https://doi.org/10.1016/j.revpalbo.2011.05.001.
Liu, X.-Y., D. Ren, and D. Yang. 2014. New transitional fossil snakeflies from China illuminate the early evolution of Raphidioptera. BMC Evolutionary Biology 14: 84. https://doi.org/10.1186/1471-2148-14-84.
Liu, X.-Y., W.-W. Zhang, S.L. Winterton, L.C.V. Breitkreuz, and M.S. Engel. 2016. Early morphological specialization for insect-spider associations in Mesozoic lacewings. Current Biology 26: 1590–1594. https://doi.org/10.1016/j.cub.2016.04.039.
Liu, X.-Y., G.-L. Shi, F.-Y. Xia, X.-M. Lu, B. Wang, and M.S. Engel. 2018. Liverwort mimesis in a Cretaceous lacewing larva. Current Biology 28: 1475–1481. https://doi.org/10.1016/j.cub.2018.03.060.
Lu, X., W. Zhang, B. Wang, M.S. Engel, and X. Liu. 2020. A new and diverse paleofauna of the extinct snakefly family Baissopteridae from the mid-Cretaceous of Myanmar (Raphidioptera). Organisms Diversity and Evolution 20: 565–595. https://doi.org/10.1007/s13127-020-00455-y.
MacLeod, E.G. 1964. A comparative morphological study of the head capsule and cervix of larval Neuroptera (Insecta). Ph.D. dissertation. Harvard University, Cambridge, Mass.
McCoy, M.W., B.M. Bolker, and C.W. Osenberg. 2006. Size correction: Comparing morphological traits among populations and environments. Oecologia 148: 547–554. https://doi.org/10.1007/s00442-006-0403-6.
Nicoli Aldini, R. 2012. Lacewings (Neuroptera) as beneficial insects in orchards: findings for plum and cherry trees in Lombardy (northern Italy). IOBC/WPRS Bulletin [=bulletin OILB/SROP] 74: 203–208.
Pérez-de la Fuente, R., E. Peñalver, X. Delclòs, and M.S. Engel. 2012a. Snakefly diversity in Early Cretaceous amber from Spain (Neuropterida, Raphidioptera). ZooKeys 204: 1–40. https://doi.org/10.3897/zookeys.204.2740.
Pérez-de la Fuente, R., X. Delclòs, E. Peñalver, M. Speranza, J. Wierzchos, C. Ascaso, and M.S. Engel. 2012b. Early evolution and ecology of camouflage in insects. Proceedings of the National Academy of Sciences 109: 21414–21419. https://doi.org/10.1073/pnas.1213775110.
Pérez-de la Fuente, R., X. Delclos, E. Penalver, and M.S. Engel. 2016. A defensive behavior and plant-insect interaction in Early Cretaceous amber–the case of the immature lacewing Hallucinochrysa diogenesi. Arthropod Structure and Development 45: 133–139. https://doi.org/10.1016/j.asd.2015.08.002.
Pérez-de la Fuente, R., M.S. Engel, X. Delclòs, and E. Penalver. 2020. Straight-jawed lacewing larvae (Neuroptera) from Lower Cretaceous Spanish amber, with an account on the known amber diversity of neuropterid immatures. Cretaceous Research 106: 104200. https://doi.org/10.1016/j.cretres.2019.104200.
Perrichot, V., and M.S. Engel. 2007. Early Cretaceous snakefly larvae in amber from Lebanon, Myanmar, and France (Raphidioptera). American Museum Novitates 3598: 1–11. https://doi.org/10.1206/0003-0082(2007)3598[1:ecslia]2.0.co;2.
Resh, V.H., and R.T. Cardé. 2003. Encyclopedia of Insects. Amsterdam and Boston: Academic Press.
Rohlf, F.J., and F.L. Bookstein. 1987. A comment on shearing as a method for “size correction.” Systematic Zoology 36: 356–367. https://doi.org/10.2307/2413400.
Saltin, B.D., C. Haug, and J.T. Haug. 2016. How metamorphic is holometabolous development? Using microscopical methods to look inside the scorpionfly (Panorpa) pupa (Mecoptera, Panorpidae). Spixiana 39: 105–118.
Scheven, J. 2004. Bernstein-Einschlüsse: Eine untergegangene Welt bezeugt die Schöpfung, Erinnerungen an die Welt vor der Sintflut. Hofheim a.T.: Kuratorium Lebendige Vorwelt e.V.
Schröder, C. 1928. Handbuch der Entomologie. Jena: Gustav Fischer.
Sedlag, U. 1986. Insekten Mitteleuropas. München: Ferdinand Enke.
Stresemann, E. 1989. Exkursionsfauna von Deutschland, Wirbellose. Insekten - Erster Halbband (Wirbellose Band. II, 1). Jena: Spektrum Akademischer Verlag.
Townsend, L.H. 1939. Lacewings and their allies. Scientific Monthly (New York) 48: 350–357.
Wachmann, E., and C. Saure. 1997. Netzflügler, Schlamm- und Kamelhalsfliegen: Beobachtung – Lebensweise. Augsburg: Naturbuch Verlag.
Wang, B., F.Y. Xia, M.S. Engel, V. Perrichot, G.L. Shi, H.C. Zhang, J. Chen, E.A. Jarzembowski, T. Wappler, and J. Rust. 2016. Debris-carrying camouflage among diverse lineages of Cretaceous insects. Science Advances 2: e1501918. https://doi.org/10.1126/sciadv.1501918.
Webster, M., and M.L. Zelditch. 2005. Evolutionary modifications of ontogeny: Heterochrony and beyond. Paleobiology 31: 354–372. https://doi.org/10.1666/0094-8373(2005)031[0354:emooha]2.0.co;2.
Weitschat, W., and W. Wichard. 1998. Atlas der Pflanzen und Tiere im baltischen Bernstein. München: Friedrich Pfeil.
Weitschat, W., and W. Wichard. 2002. Atlas of Plants and Animals in Baltic Amber. München: Friedrich Pfeil.
Winterton, S.L., A.R. Lemmon, J.P. Gillung, I.J. Garzon, D. Badano, D.K. Bakkes, L.C.V. Breitkreuz, M.S. Engel, E. Moriarity Lemmon, X.-Y. Liu, R.J.P. Machado, J.H. Skevington, and J.D. Oswald. 2018. Evolution of lacewings and allied orders using anchored phylogenomics (Neuroptera, Megaloptera, Raphidioptera). Systematic Entomology 43: 330–354. https://doi.org/10.1111/syen.12278.
Woglum, R.S., and E.A. McGregor. 1958. Observations on the life history and morphology of Agulla bractea Carpenter (Neuroptera: Raphidiodea: Raphidiidae). Annals of the Entomological Society of America 51: 129–141. https://doi.org/10.1093/aesa/51.2.129.
Woglum, R.S., and E.A. McGregor. 1959. Observations on the life history and morphology of Agulla astuta (Banks) (Neuroptera: Raphidiodea: Raphidiidae). Annals of the Entomological Society of America 52: 489–502. https://doi.org/10.1093/aesa/52.5.489.
Xia, F., G. Yang, Q. Zhang, G. Shi, and B. Wang. 2015. Amber: Life Through Time and Space. Beijing: Science Press.
Yahya, H. 2007. Atlas of Creation, vol. II. Istanbul: Global Publishing.
Yahya, H. 2008. Atlas der Schöpfung, vol. III. Istanbul: Global Publishing.
Yang, A.S. 2001. Modularity, evolvability, and adaptive radiations: A comparison of hemi- and holometabolous insects. Evolution and Development 3: 59–72.
Zhang, W.W. 2017. Frozen Dimensions. The Fossil Insects and Other Invertebrates in Amber. Chongqing: Chongqing University Press.
Acknowledgements
Mike Reich is thanked for handling the manuscript. We are grateful to one anonymous reviewer for helpful comments. We thank the various curators for providing access to specimens in their care: Martin Husemann, Ulrich Kotthoff (both Centrum für Naturkunde (CeNak), Leibniz-Institut zur Analyse des Biodiversitätswandels (LIB), Hamburg), Alexander Gehler (Geoscience Museum Göttingen), Mónica Solórzano Kraemer (Senckenberg Museum Frankfurt). We are grateful to all individuals providing images of extant and fossil snakefly larvae used in this study, which significantly increased our dataset. J. Matthias Starck is thanked for long-time support. We thank all people providing free software. This is LEON publication #21.
Funding
Open Access funding enabled and organized by Projekt DEAL. This work was funded by Volkswagen Foundation (Lichtenberg professorship); Deutsche Forschungsgemeinschaft (DFG HA 6300/6–1); University of Kansas (Michener Fund in Entomology); Ludwig-Maximilians-Universität München (Lehre@LMU).
Author information
Authors and Affiliations
Contributions
Conceptualization: JTH, MSE, CH; methodology: JTH, MSE, PMS, GTH, PM, CH; formal analysis and investigation: JTH, MSE, PMS, GTH, PM, CH; writing—original draft preparation: JTH, MSE, CH; writing—review and editing: JTH, MSE, PMS, GTH, PM, CH; funding acquisition: JTH, MSE; resources: JTH, PM.
Corresponding author
Ethics declarations
Conflict of interest
The authors declare that they have no conflicts of interest and no competing interests.
Additional information
Handling Editor: Mike Reich.
Supplementary Information
Below is the link to the electronic supplementary material.
12542_2022_609_MOESM1_ESM.doc
Supplementary file1 Supplementary Text 1 Overview of all specimens used in this study with short descriptions (DOC 37 KB)
12542_2022_609_MOESM2_ESM.tif
Supplementary file2 Supplementary Figure 1 Screenshots of the principal component analysis (PCA) performed in PAST (TIF 339 KB)
12542_2022_609_MOESM3_ESM.xls
Supplementary file3 Supplementary Table 1 List of all specimens used in this study including measurements and calculated ratios. al antenna length, bl body length, hl head length, hw head width, n.a. not applicable, pl pronotum length, pw pronotum width, rtl remaining trunk length, rtw remaining trunk width (XLS 55 KB)
Rights and permissions
Open Access This article is licensed under a Creative Commons Attribution 4.0 International License, which permits use, sharing, adaptation, distribution and reproduction in any medium or format, as long as you give appropriate credit to the original author(s) and the source, provide a link to the Creative Commons licence, and indicate if changes were made. The images or other third party material in this article are included in the article's Creative Commons licence, unless indicated otherwise in a credit line to the material. If material is not included in the article's Creative Commons licence and your intended use is not permitted by statutory regulation or exceeds the permitted use, you will need to obtain permission directly from the copyright holder. To view a copy of this licence, visit http://creativecommons.org/licenses/by/4.0/.
About this article
Cite this article
Haug, J.T., Engel, M.S., Mendes dos Santos, P. et al. Declining morphological diversity in snakefly larvae during last 100 million years. PalZ 96, 749–780 (2022). https://doi.org/10.1007/s12542-022-00609-7
Received:
Accepted:
Published:
Issue Date:
DOI: https://doi.org/10.1007/s12542-022-00609-7