Abstract
The problem of the special rotational deformation interacting with an internal crack is studied by developing a theoretical model of deformed nanocrystalline materials. Using the complex variable method of Muskhelishvili and the conformal mapping technique, the expressions of complex potentials and stress fields are obtained analytically. The stress intensity factors (SIFs) near the crack tips and the critical SIFs for the first lattice dislocation emission from the branched crack tip are calculated. The effects of important parameters such as grain size, the length of internal crack, and the angle between the main crack and its branched crack on the critical SIFs for dislocation emission are evaluated in detail. As a result, the special rotational deformation has great influence on the growth of internal crack and the emission of lattice dislocations from the branched crack tip. The disclination quadrupole produced by the special rotational deformation will shield the branched crack tip under a certain condition. Moreover, when the main crack approaches its branched crack, it will stop the emission of lattice dislocations from the branched crack tip.
Similar content being viewed by others
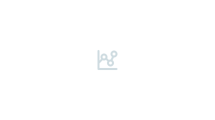
References
Kuntz, J.D., Zhan, G.-D., Mukherjee, A.K.: Nanocrystalline-matrix ceramic composites for improved fracture toughness. MRS Bull. 29(01), 22–27 (2004). doi:10.1557/mrs2004.12
Ovid’ko, I.: Deformation and diffusion modes in nanocrystalline materials. Int. Mater. Rev. 50(2), 65–82 (2005). doi:10.1179/174328005X14294
Wolf, D., Yamakov, V., Phillpot, S., Mukherjee, A., Gleiter, H.: Deformation of nanocrystalline materials by molecular-dynamics simulation: relationship to experiments? Acta Mater. 53(1), 1–40 (2005). doi:10.1016/j.actamat.2004.08.045
Dao, M., Lu, L., Asaro, R., De Hosson, J.T.M., Ma, E.: Toward a quantitative understanding of mechanical behavior of nanocrystalline metals. Acta Mater. 55(12), 4041–4065 (2007). doi:10.1016/j.actamat.2007.01.038
Mukhopadhyay, A., Basu, B.: Consolidation–microstructure–property relationships in bulk nanoceramics and ceramic nanocomposites: a review. Int. Mater. Rev. 52(5), 257–288 (2007). doi:10.1179/174328007X160281
Barai, P., Weng, G.J.: Mechanics of creep resistance in nanocrystalline solids. Acta Mech. 195(1–4), 327–348 (2008). doi:10.1007/s00707-007-0558-1
Barai, P., Weng, G.J.: The competition of grain size and porosity in the viscoplastic response of nanocrystalline solids. Int. J. Plast. 24(8), 1380–1410 (2008). doi:10.1016/j.ijplas.2007.09.010
Gurtin, M.E., Anand, L.: Nanocrystalline grain boundaries that slip and separate: a gradient theory that accounts for grain-boundary stress and conditions at a triple-junction. J. Mech. Phys. Solids 56(1), 184–199 (2008). doi:10.1016/j.jmps.2007.09.001
Wei, Y., Bower, A.F., Gao, H.: Enhanced strain-rate sensitivity in fcc nanocrystals due to grain-boundary diffusion and sliding. Acta Mater. 56(8), 1741–1752 (2008). doi:10.1016/j.actamat.2007.12.028
Morozov, N., Ovid’ko, I., Petrov, Y.V., Sheinerman, A.: Generation and converence of nanocracks in nanocrystalline materials deformed by grain boundary sliding. Rev. Adv. Mater. Sci. 19, 63–72 (2009)
Weng, G.J.: A homogenization scheme for the plastic properties of nanocrystalline materials. Rev. Adv. Mater. Sci. 19, 41–62 (2009)
Aifantis, E.: Deformation and failure of bulk nanograined and ultrafine-grained materials. Mater. Sci. Eng. A 503(1), 190–197 (2009). doi:10.1016/j.msea.2008.04.085
Wu, M.: Analysis of dislocation wall formation in a disclinated nanograin. Int. J. Plast. 26(6), 794–805 (2010). doi:10.1016/j.ijplas.2009.10.007
Yang, F., Yang, W.: Crack growth versus blunting in nanocrystalline metals with extremely small grain size. J. Mech. Phys. Solids 57(2), 305–324 (2009). doi:10.1016/j.jmps.2008.10.011
Figueiredo, R.B., Kawasaki, M., Langdon, T.G.: The mechanical properties of ultrafine-grained metals at elevated temperatures. Rev. Adv. Mater. Sci. 19(1/2), 1–12 (2009)
Guo, X., Weng, G., Soh, A.: Ductility enhancement of layered stainless steel with nanograined interface layers. Comput. Mater. Sci. 55, 350–355 (2012). doi:10.1016/j.commatsci.2011.11.014
Pei, Y., Galvan, D., De Hosson, J.T.M.: Nanostructure and properties of TiC/aC: H composite coatings. Acta Mater. 53(17), 4505–4521 (2005). doi:10.1016/j.actamat.2005.05.045
Kaminskii, A., Akchurin, M.S., Gainutdinov, R., Takaichi, K., Shirakava, A., Yagi, H., Yanagitani, T., Ueda, K.: Microhardness and fracture toughness of \(\text{ Y }_2\text{ O }_3\)-and \(\text{ Y }_3\text{ Al }_5\text{ O }_12\)-based nanocrystalline laser ceramics. Crystallogr. Rep. 50(5), 869–873 (2005). doi:10.1134/1.2049410
Bobylev, S., Morozov, N., Ovid’ko, I.: Cooperative grain boundary sliding and nanograin nucleation process in nanocrystalline, ultrafine-grained, and polycrystalline solids. Phys. Rev. B Condens. Matter 84(9), 094103 (2011). doi:10.1103/PhysRevB.84.094103
Juan, P.-A., Berbenni, S., Capolungo, L.: Prediction of internal stresses during growth of first-and second-generation twins in Mg and Mg alloys. Acta Mater. 60(2), 476–486 (2012). doi:10.1016/j.actamat.2011.10.018
Morozov, N., Ovid’ko, I., Sheinerman, A., Aifantis, E.: Special rotational deformation as a toughening mechanism in nanocrystalline solids. J. Mech. Phys. Solids 58(8), 1088–1099 (2010). doi:10.1016/j.jmps.2010.04.003
Ovid’ko, I., Sheinerman, A.: Nanoscale rotational deformation in solids at high stresses. Appl. Phys. Lett. 98(18), 181909 (2011). doi:10.1063/1.3587637
Mukherjee, A.K.: An examination of the constitutive equation for elevated temperature plasticity. Mater. Sci. Eng. A 322(1), 1–22 (2002). doi:10.1016/S0921-5093(01)01115-7
Murayama, M., Howe, J., Hidaka, H., Takaki, S.: Atomic-level observation of disclination dipoles in mechanically milled, nanocrystalline Fe. Science 295(5564), 2433–2435 (2002). doi:10.1126/science.1067430
Zizak, I., Darowski, N., Klaumünzer, S., Schumacher, G., Gerlach, J.W., Assmann, W.: Ion-beam-induced collective rotation of nanocrystals. Phys. Rev. Lett. 101(6), 065503 (2008). doi:10.1103/PhysRevLett.101.065503
Shan, Z., Stach, E., Wiezorek, J., Knapp, J., Follstaedt, D., Mao, S.: Grain boundary-mediated plasticity in nanocrystalline nickel. Science 305(5684), 654–657 (2004). doi:10.1126/science.1098741
Szlufarska, I., Nakano, A., Vashishta, P.: A crossover in the mechanical response of nanocrystalline ceramics. Science 309(5736), 911–914 (2005). doi:10.1126/science.1114411
Shimokawa, T., Nakatani, A., Kitagawa, H.: Grain-size dependence of the relationship between intergranular and intragranular deformation of nanocrystalline Al by molecular dynamics simulations. Phys. Rev. B Condens. Matter 71(22), 224110 (2005). doi:10.1103/PhysRevB.71.224110
Latapie, A., Farkas, D.: Molecular dynamics investigation of the fracture behavior of nanocrystalline \(\alpha \)-Fe. Phys. Rev. B Condens. Matter 69(13), 134110 (2004). doi:10.1103/PhysRevB.69.134110
Bobylev, S., Mukherjee, A., Ovid’ko, I.: Transition from plastic shear into rotation deformation mode in nanocrystalline metals and ceramics. Rev. Adv. Mater. Sci. 19, 103–113 (2009)
Ovid’ko, I., Sheinerman, A.: Special rotational deformation in nanocrystalline metals and ceramics. Scr. Mater. 59(1), 119–122 (2008). doi:10.1016/j.scriptamat.2008.02.047
Joshi, S.P., Ramesh, K.: Stability map for nanocrystalline and amorphous materials. Phys. Rev. Lett. 101(2), 025501 (2008). doi:10.1103/PhysRevLett.101.025501
Liu, Y., Zhou, J., Wang, L., Zhang, S., Wang, Y.: Grain size dependent fracture toughness of nanocrystalline materials. Mater. Sci. Eng. A 528(13), 4615–4619 (2011). doi:10.1016/j.msea.2011.02.056
Liu, Y., Zhou, J., Shen, T., Hui, D.: Grain rotation dependent fracture toughness of nanocrystalline materials. Mater. Sci. Eng. A 528(25), 7684–7687 (2011). doi:10.1016/j.msea.2011.06.035
Feng, H., Fang, Q., Liu, Y., Chen, C.: Nanoscale rotational deformation effect on dislocation emission from an elliptically blunted crack tip in nanocrystalline materials. Int. J. Solids Struct. 51(2), 352–358 (2014). doi:10.1016/j.ijsolstr.2013.10.008
Feng, H., Fang, Q.H., Zhang, L.C., Liu, Y.W.: Special rotational deformation and grain size effect on fracture toughness of nanocrystalline materials. Int. J. Plast. 42(4), 50–64 (2013). doi:10.1016/j.ijplas.2012.09.015
Fang, Q., Feng, H., Liu, Y., Lin, S., Zhang, N.: Special rotational deformation effect on the emission of dislocations from a crack tip in deformed nanocrystalline solids. Int. J. Solids Struct. 49(11), 1406–1412 (2012). doi:10.1016/j.ijsolstr.2012.02.026
Yu, M., Fang, Q., Feng, H., Liu, Y.: Effect of special rotational deformation on dislocation emission from interface collinear crack tip in nanocrystalline bi-materials. Acta Mech. (2016). doi:10.1007/s00707-016-1604-7
Muskhelishvili, N.I.: Some Basic Problems of the Mathematical Theory of Elasticity, vol. 1. Springer, Berlin (1977)
Sih, G.: Stress distribution near internal crack tips for longitudinal shear problems. J. Appl. Mech. 32(1), 51–58 (1965)
Romanov, A., Vladimirov, V.: Disclinations in crystalline solids. In: Nabarro, F.R.N. (ed.) Dislocations in Solids, vol. 9. North-Holland, Amsterdam (1992)
Zhang, T.-Y., Tong, P., Ouyang, H., Lee, S.: Interaction of an edge dislocation with a wedge crack. J. Appl. Phys. 78(8), 4873 (1995). doi:10.1063/1.359775
Hao-Peng, S., Qi-Hong, F., You-Wen, L.: Elastic behaviour of a wedge disclination dipole near a sharp crack emanating from a semi-elliptical blunt crack. Chin. Phys. B 19(5), 056102 (2010). doi:10.1088/1674-1056/19/5/056102
Fang, Q., Liu, Y.: Size-dependent interaction between an edge dislocation and a nanoscale inhomogeneity with interface effects. Acta Mater. 54(16), 4213–4220 (2006). doi:10.1016/j.actamat.2006.05.012
Fang, Q., Liu, Y., Jin, B., Wen, P.: Effect of interface stresses on the image force and stability of an edge dislocation inside a nanoscale cylindrical inclusion. Int. J. Solids Struct. 46(6), 1413–1422 (2009). doi:10.1016/j.ijsolstr.2008.11.013
Hirth, J.P., Lothe, J.: Theory of Dislocations. Wiley, New York (1982)
Neuber, H.: Theory of Notch Stresses. JW Edwards, Ann Arbor (1946)
Irwin, G.R.: Analysis of stresses and strains near the end of a crack traversing a plate. SPIE Milest. Ser. MS 137, 167–170 (1997)
Rice, J.R., Thomson, R.: Ductile versus brittle behaviour of crystals. Philos. Mag. 29(1), 73–97 (1974). doi:10.1080/14786437408213555
Huang, M., Li, Z.: Dislocation emission criterion from a blunt crack tip. J. Mech. Phys. Solids 52(9), 1991–2003 (2004). doi:10.1016/j.jmps.2004.03.003
Author information
Authors and Affiliations
Corresponding author
Rights and permissions
About this article
Cite this article
He, T., Xiao, W., Zhang, Y. et al. Effect of special rotational deformation on the dislocation emission from a branched crack tip in deformed nanocrystalline materials. Acta Mech 228, 823–836 (2017). https://doi.org/10.1007/s00707-016-1742-y
Received:
Revised:
Published:
Issue Date:
DOI: https://doi.org/10.1007/s00707-016-1742-y