Abstract
The main intention of this article is that new techniques of existence theory are used to derive some required criteria pertinent to a given fractional multi-term problem and its inclusion version. In such an approach, we do our research on a fractional integral equation corresponding to the mentioned BVPs. In more precise words, by virtue of this integral equation, we construct new operators which belong to a special category of functions named α-admissible and α-ψ-contraction maps coupled with operators having (AEP)-property. Next, by considering some new properties on the existing Banach space having properties (B) and \((C_{\alpha })\), our argument for ensuring the existence of solutions is completed. In addition, we also add two simulative examples to review our findings by a numerical view.
Similar content being viewed by others
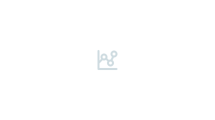
1 Introduction
As is well known, fractional calculus (FC), for the sake of its higher accuracy than that of the integer one, is an essential topic that is considered as a strong tool in description of natural laws in many branches of science including electrical networks, rheology, biology, dynamical systems, biophysics coupled with many mathematical modelings formulated by a vast diversity of fractional operators; review for details [1–8].
Among theoretical concepts and methods, the theory of the existence of solution on the large domain of different fractional constructions including differential equations and inclusions has gained the attention of many mathematicians and relevant researchers. Most of them have focused on applying Caputo, Riemann–Liouville (RL), Hadamard, and many other derivation operators to illustrate the underlying fractional differential equations. Along with these structures, we observe different published articles recently in which the existence of solutions is derived for interesting categories of fractional local or nonlocal, multi-term, multi-point, multi-strip, multi-order fractional differential equations; see [9–21].
In 2018, Tariboon et al. [22] discussed a new category of separated sequential BVP in the context of mixed Hadamard and Caputo operators as follows:
where \({}^{\mathcal{C}}\mathfrak{D}_{m}^{\sigma _{1}^{*}}\) and \({}^{\mathcal{H}}\mathfrak{D}_{m}^{\sigma _{2}^{*}}\) stand for two different kinds of derivatives named Caputo and Hadamard of order \(\sigma _{1}^{*} , \sigma _{2}^{*} \in (0,1]\), \(a_{1}, a_{2}, a_{3}, a_{4}\in \mathbb{R}\) and \(\Phi ^{*}: \mathbb{J}^{*} \times \mathbb{R} \rightarrow \mathbb{R}\) is continuous. In that article, some standard methods pertinent to fixed point theory are used for obtaining the existence results.
In 2019, Mahmudov and Al-Khateeb [23] studied a novel sequential system of coupled BVPs with nonseparated conditions as follows:
in which a derivative operator \({}^{\mathcal{C}}\mathfrak{D}_{0^{+}}^{j}\) is of Caputo type with \(j \in \{ \sigma _{1}^{*} , \sigma _{2}^{*} \} \subseteq (1,2] \), \(\mu \in \mathbb{R}_{> 0}\), and \(a_{l} , b_{l} \in \mathbb{R}\) with \(l\in \{ 1,2\}\). Stability analysis and existence notions are two main goals of that work which are investigated by means of fixed point methods.
In 2020, Mohammadi et al. [24] studied a specific kind of three-point sequential inclusion BVP which has the following form:
where \(\sigma ^{*} \in (2, 3] \), \(\xi \in (0,1)\), \(\zeta _{1}, \zeta _{2} >0 \), and \({}^{\mathcal{RL}}\mathcal{I}_{0^{+}}^{\gamma } \) denotes the Riemann–Liouville integral of order \(\gamma >0\). With the help of a new analytical process, the authors explored desired outcomes in relation to solutions of a non-hybrid structure.
With the help of ideas of the above articles, we investigate and discuss new existence techniques and methods to ensure the existence of solutions for a new multi-term problem illustrated as follows:
where \({{}^{\mathcal{RL}}}\mathfrak{I}_{0}^{(\cdot )}\) and \({}^{\mathcal{C}}\mathfrak{D}_{0}^{ (\cdot )}\) denote integration and derivation operators in the sense of Riemann–Liouville and Caputo, respectively, such that \(\theta ^{*}>0\), \(0 <\sigma ^{*} < 1\), \(1<\delta ^{*}<2\). Besides, we have taken \(a_{1}, a_{2}, a_{3}, a_{4}\in \mathbb{R}^{+}\) and \(\Phi ^{*}: \mathbb{J} \times \mathbb{R}^{2} \rightarrow \mathbb{R}\) as a continuous map.
Also along with the above problem, we derive the existence of solution for the following inclusion version which takes a form as follows:
where we consider \(\mathfrak{H}\) as a multifunction on the product space \(\mathbb{J} \times \mathbb{R}^{2}\) containing different properties which will be introduced later.
By studying a wide range of published articles pertinent to the existence and uniqueness notions in the context of fractional boundary value problems, we see that many authors usually utilize some standard methods based on the famous fixed point techniques to derive desired results in relation to the existence of solutions. The novelty of our work in the current research is that we introduce a new construction of two fractional multi-term BVPs having integral conditions and then build new operators which belong to a new class of specific functions. Two main functions belonging to this class are α-admissible maps and α-ψ-contractive maps. Here, by using these functions on a space having properties (B) and \((C_{\alpha })\), we derive the existence results for both suggested BVPs (1) and (2). Besides, by virtue of the (AEP)-property for the obtained multifunction in the proof and with the help of the endpoint notion, we derive another criterion of the existence of solutions. As you will see, our techniques used for supposed problems (1) and (2) have been done in limited works, and for the first time, we apply these methods on a multi-term structures simultaneously.
The arrangement of the notions of this research are as follows: Sect. 2 is devoted to reviewing some primitive notions. In Sect. 3, by means of α-ψ-contractive functions, we prove our existence results for (1). Then, in Sect. 4, we derive other criteria stating the solution’s existence for the inclusion BVP (2) by considering the extended category of α-ψ-contractive functions on multifunctions. Two numerical examples are also given to confirm findings in Sect. 5. At last, we summarize our method and specify new directions for the future works in Sect. 6.
2 Preliminaries
At this moment, we collect and review several auxiliary and primitive concepts in the context of our methods used in this research. As you know, the concepts of the Riemann–Liouville operator and the Caputo one have an important role in fractional calculus, we recall some properties of them here.
Definition 2.1
The Riemann–Liouville integral of a map \(\upsilon : [0, + \infty ) \to \mathbb{R}\) of order \(\sigma ^{*} >0\) is defined by
if the integral exists.
Definition 2.2
Let \(n = [ \sigma ^{*} ] +1\). For a continuous map \(\upsilon : \mathbb{R}_{\geq 0} \to \mathbb{R}\), the Riemann–Liouville derivative is defined by
if the integral exists.
Definition 2.3
Let \(n = [ \sigma ^{*} ] +1\). For a real absolutely continuous function υ on \(\mathbb{R}^{\geq 0}\), the Caputo derivative is defined by
such that the integral exists.
Proposition 2.4
([27])
Let \(n-1 < \sigma ^{*} < n\). Then, for every \(\upsilon \in C^{n-1} (\mathbb{J})\), we have
for some \(c_{0}^{*} , c_{1}^{*} , \dots , c_{n-1}^{*} \in \mathbb{R}\).
Assuming \((\mathcal{M},\|\cdot \|)\) as a normed space, we mean by \({\mathcal{P}}_{CL}( \mathcal{M} )\), \({\mathcal{P}}_{BN}( \mathcal{M} )\), \({\mathcal{P}}_{CP}( \mathcal{M} )\), and \({\mathcal{P}}_{CV}( \mathcal{M} )\) the collection of all closed, bounded, compact, and convex subsets of \(\mathcal{M} \), respectively.
Definition 2.5
([28])
Consider \(\upsilon : \mathbb{R} \to \mathbb{R}\) as a real-valued function and v as a multifunction.
-
(i)
\(\mathfrak{H}\) is u.s.c on \(\mathcal{M} \) if \(\mathfrak{H}(\upsilon ^{*})\in \mathcal{P}_{CL}(\mathcal{M})\) for any \(\upsilon ^{*} \in \mathcal{M} \) and also a neighborhood \(\mathfrak{N}_{0}^{*}\) of \(\upsilon ^{*}\) exists subject to \(\mathfrak{H}(\mathfrak{N}_{0}^{*}) \subseteq \mathbb{V}\) for \(\mathbb{V} \subseteq \mathcal{M}\) in which \(\mathbb{V}\) is an arbitrary open set.
-
(ii)
A real-valued map \(\upsilon : \mathbb{R} \to \mathbb{R}\) is upper semi-continuous such that \(\lim \sup_{n\to \infty } \upsilon (\varsigma _{n})\leq \upsilon ( \varsigma )\) for each \(\{ \varsigma _{n} \}_{n\geq 1}\) with \(\varsigma _{n} \to \varsigma \).
Definition 2.6
([28])
A metric attributed to Pompeiu–Hausdorff \(\mathcal{H}_{d} : ( \mathcal{P}(\mathcal{M}) )^{2} \to \mathbb{R} \cup \{\infty \}\) is defined as
where d is considered the metric of \(\mathcal{M}\) and also \(d(\mathcal{Q}_{1}^{*},q_{2}^{*}) = \inf_{q_{1}^{*}\in \mathcal{Q}_{1}^{*}}d(q_{1}^{*},q_{2}^{*})\) and \(d(q_{1}^{*},\mathcal{Q}_{2}^{*}) = \inf_{q_{2}^{*}\in \mathcal{Q}_{2}^{*}} d(q_{1}^{*},q_{2}^{*})\).
Definition 2.7
([28])
For \(\mathfrak{H} : \mathcal{M} \to {\mathcal{P}}_{CL} (\mathcal{M})\) and \(\upsilon _{1}, \upsilon _{2} \in \mathcal{M}\), let the following inequality hold:
Then \(\mathfrak{H}\) is said to be: (H1) a Lipschitz map if \(\ell > 0\); (H2) a contraction if \(0 < \ell < 1\).
Definition 2.8
([28])
-
(i)
\(\mathfrak{H}: \mathbb{J}\times \mathbb{R} \to {\mathcal{P}}( \mathbb{R})\) is Caratheodory if \(z \mapsto \mathfrak{H}(z ,\upsilon )\) is measurable for any \(\upsilon \in \mathbb{R}\) and \(\upsilon \mapsto \mathfrak{H}(a ,\upsilon ) \) is u.s.c for a.e. \(z\in \mathbb{J}\).
-
(ii)
A Caratheodory multifunction \(\mathfrak{H}: \mathbb{J}\times \mathbb{R} \to \mathcal{P}(\mathbb{R})\) is \(L^{1}\)-Caratheodory if, for any \(\zeta >0\), \(\kappa _{\zeta }\in L^{1} (\mathbb{J}, \mathbb{R}_{+})\) exists subject to \(\Vert \mathfrak{H}(z ,\upsilon ) \Vert =\sup_{z \in \mathbb{J}}\{| \omega |:\omega \in \mathfrak{H}(z ,\upsilon )\}\leq \kappa _{\zeta }(z)\) for all \(| \upsilon |\leq \zeta \) and for almost all \(z \in \mathbb{J}\).
Definition 2.9
([28])
Consider \(\mathcal{M}\) as a metric space. Then
-
(i)
\(\upsilon \in \mathcal{M}\) is an endpoint of \(\mathfrak{H} :\mathcal{M}\to \mathcal{P}(\mathcal{M})\) if \(\mathfrak{H}\upsilon =\{ \upsilon \}\).
-
(ii)
\(\mathfrak{H}\) has an approximate endpoint property (or (AEP)-property) if
$$ \inf_{w\in \mathcal{M}}\sup_{\upsilon \in \mathfrak{H}w}d(w, \upsilon )=0. $$
To meet the argumentative purposes of this paper, we shall apply a specific set of functions and properties pertinent to them. For the first time, Samet, Vetro, and Vetro [29] constructed such a category of mappings in 2012.
Definition 2.10
([29])
Consider all nondecreasing maps \(\psi : \mathbb{R}_{\geq 0}\to \mathbb{R}_{\geq 0}\) belonging to class Π such that, for all \(z>0\), \(\sum_{j=1}^{\infty }\psi ^{j}(z)<\infty \) and \(\psi (z)< z\). Let \(\Phi ^{*}: \mathcal{M}\to \mathcal{M}\) and \(\alpha : \mathcal{M}^{2} \to \mathbb{R}_{\geq 0}\). Then
-
(i)
\(\Phi ^{*}\) is an α-ψ-contraction if, for \(\upsilon _{1}, \upsilon _{2}\in \mathcal{M}\),
$$ \alpha (\upsilon _{1},\upsilon _{2}) d\bigl(\Phi ^{*} \upsilon _{1}, \Phi ^{*} \upsilon _{2}\bigr)\leq \psi \bigl(d(\upsilon _{1}, \upsilon _{2})\bigr). $$ -
(ii)
\(\Phi ^{*}\) is α-admissible if \(\alpha (\upsilon _{1},\upsilon _{2}) \geq 1\) gives \(\alpha (\Phi ^{*}\upsilon _{1},\Phi ^{*}\upsilon _{2}) \geq 1\).
-
(iii)
\(\mathcal{M}\) has the property (B) if, for every sequence \(\{\upsilon _{n}\}_{n\geq 1}\) of \(\mathcal{M}\) with \(\alpha (\upsilon _{n},\upsilon _{n+1})\geq 1\) and \(\upsilon _{n}\to \upsilon \), the inequality \(\alpha (\upsilon _{n},\upsilon )\geq 1\) is satisfied for all \(n \geq 1\).
The second category of special maps is an extension of the previous one which was constructed by Mohammadi et al. one year later [30].
Definition 2.11
([30])
Consider all nondecreasing maps \(\psi : \mathbb{R}_{\geq 0}\to \mathbb{R}_{\geq 0}\) belonging to class Π such that, for all \(z>0\), \(\sum_{j=1}^{\infty }\psi ^{j}(z)<\infty \) and \(\psi (z)< z\). Let \(\mathfrak{H}: \mathcal{M}\to \mathcal{P}(\mathcal{M})\) and \(\alpha : \mathcal{M}^{2} \to \mathbb{R}_{\geq 0}\). Then
-
(i)
\(\mathfrak{H}: \mathcal{M} \to \mathcal{P}_{CL,BN}(\mathcal{M})\) is an α-ψ-contraction if, for each \(\upsilon _{1}, \upsilon _{2}\in \mathcal{M}\),
$$ \alpha (\upsilon _{1},\upsilon _{2}) \mathcal{H}_{d} (\mathfrak{H} \upsilon _{1}, \mathfrak{H} \upsilon _{2})\leq \psi \bigl(d(\upsilon _{1}, \upsilon _{2})\bigr). $$ -
(ii)
\(\mathfrak{H}\) is α-admissible if, for each \(\upsilon _{1}\in \mathcal{M}\) and \(\upsilon _{2}\in \mathfrak{H}\upsilon _{1}\), the inequality \(\alpha (\upsilon _{1},\upsilon _{2})\geq 1\) gives \(\alpha ( \upsilon _{2},\upsilon _{3})\geq 1\) for each \(\upsilon _{3}\in \mathfrak{H}\upsilon _{2}\).
-
(iii)
\(\mathcal{M}\) has the property \((C_{\alpha })\) if, for every sequence \(\{ \upsilon _{n} \}_{n \geq 1} \) of \(\mathcal{M}\) with \(\upsilon _{n}\to \upsilon \) and \(\alpha (\upsilon _{n} , \upsilon _{n+1}) \geq 1\), a subsequence \(\{ \upsilon _{n_{k}} \}\) of \(\{ \upsilon _{n} \}\) exists such that \(\alpha (\upsilon _{n_{k}}, \upsilon )\geq 1\) for all \(k\in \mathbb{N}\).
Next theorems are considered as the basis of our arguments until the end of this research.
Theorem 2.12
([29])
Consider the complete metric space \((\mathcal{M},d)\), \(\psi \in \Pi \), \(\alpha : \mathcal{M}^{2}\to \mathbb{R}\) and \(\Phi ^{*}: \mathcal{M} \to \mathcal{M}\). Assume that:
-
(i)
\(\Phi ^{*}\) is α-admissible and α-ψ-contraction;
-
(ii)
\(\alpha (\upsilon _{0}, \Phi ^{*}\upsilon _{0} )\geq 1\) for some \(\upsilon _{0} \in \mathcal{M}\);
-
(iii)
\(\mathcal{M}\) has the property (B).
In that case, \(\Phi ^{*}\) has a fixed point.
Theorem 2.13
([31])
Assuming \(\mathcal{M}\) as a Banach space, let \(\mathbb{A} \neq \emptyset \) belong to \(\mathcal{P}_{CL,BN,CV}(\mathcal{M})\). For \(\mathfrak{T}_{1}\) and \(\mathfrak{T}_{1}\) defined on \(\mathbb{A}\), the following assertions hold:
-
(i)
\(\mathfrak{T}_{1} \upsilon +\mathfrak{T}_{2} \upsilon ' \in \mathbb{A}\) for \(\upsilon , \upsilon '\in \mathbb{A}\);
-
(ii)
\(\mathfrak{T}_{1}\) is compact-continuous;
-
(iii)
\(\mathfrak{T}_{2}\) is a contraction.
Then \(\upsilon _{*}\in \mathbb{A}\) exists such that \(\upsilon _{*}=\mathfrak{T}_{1}\upsilon _{*}+\mathfrak{T}_{2} \upsilon _{*}\).
Theorem 2.14
([30])
Consider the complete metric space \((\mathcal{M},d)\), \(\psi \in \Pi \), \(\alpha : \mathcal{M}^{2}\to \mathbb{R}_{\geq 0}\), and \(\mathfrak{H}: \mathcal{M} \to \mathcal{P}_{CL,BN}(\mathcal{M})\). Assume that:
-
(i)
\(\mathfrak{H}\) is α-admissible and α-ψ-contraction;
-
(ii)
\(\alpha (\upsilon _{0}, \upsilon _{1} )\geq 1\) for some \(\upsilon _{0} \in \mathcal{M}\) and \(\upsilon _{1} \in \mathfrak{H}\upsilon _{0}\);
-
(iii)
\(\mathcal{M}\) has the property \((C_{\alpha })\).
In that case, \(\mathfrak{H}\) has a fixed point.
Theorem 2.15
([28])
Consider \((\mathcal{M},d)\) as a complete metric space. Assume that:
-
(i)
\(\psi \in \Pi \) is u.s.c coupled with \(\liminf_{z\to \infty }(z-\psi (z))>0\) for \(z>0\);
-
(ii)
\(\mathfrak{H} : \mathcal{M} \to \mathcal{P}_{CL,BN}(\mathcal{M})\) satisfies the property \(\mathcal{H}_{d}(\mathfrak{H} \upsilon _{1},\mathfrak{H} \upsilon _{2}) \leq \psi (d(\upsilon _{1}, \upsilon _{2}))\) for \(\upsilon _{1}, \upsilon _{2} \in \mathcal{M}\).
Then \(\mathfrak{H}\) has a unique endpoint if and only if \(\mathfrak{H}\) has the (AEP)-property.
3 Existence criteria for problem (1)
In the present situation of the current research, we start to follow the essential deductions on the existence notion for possible solutions of problem (1) through existing nonlinear techniques in the theory of fixed points of an assumed operator. To begin the desired approach, the set \(\mathcal{M}=\{\upsilon (z): \upsilon (z), {}^{\mathcal{C}} \mathfrak{D}_{0}^{1}\upsilon (z)\in C(\mathbb{J}, \mathbb{R})\}\) regarded as a Banach space is equipped with \(\|\upsilon \|_{\mathcal{M}}=\sup_{z\in \mathbb{J}}|\upsilon (z)|+ \sup_{z\in \mathbb{J}}|{}^{\mathcal{C}}\mathfrak{D}_{0}^{1}\upsilon (z)|\) for all \(\upsilon \in \mathcal{M}\). In the next lemma, the solution of the supposed problem (1) is exhibited in the framework of an integral equation which will be useful for our subsequent arguments.
Lemma 3.1
Let \(\theta ^{*}>0\), \(0 <\sigma ^{*} < 1\), \(1<\delta ^{*}<2\), \(a_{1}, a_{2}, a_{3}, a_{4} \in \mathbb{R}^{+}\), and \(\phi \in C(\mathbb{J},\mathbb{R})\). In that case, the solution of the fractional BVP which takes the structure
is illustrated as
where
are nonzero constants.
Proof
Let \(\upsilon (z)\) be a solution of (3). With due attention to Proposition 2.4, three constants \(c_{0}, c_{1}, c_{2}\in \mathbb{R}\) exist such that
Using \(\upsilon (0)=0\), we have \(c_{0}=0\), so (6) becomes
Now, we compute
and
By using the second boundary condition \(a_{1}^{\mathcal{C}}\mathfrak{D}_{0}^{1} \upsilon (0)+a_{2} {{}^{ \mathcal{C}}}\mathfrak{D}_{0}^{\delta ^{*}} \upsilon (1)=0\), this gives
By using the third boundary condition \(a_{3}^{\mathcal{C}}\mathfrak{D}_{0}^{2} \upsilon (0)+a_{4} {{}^{ \mathcal{RL}}}\mathfrak{I}_{0}^{\theta ^{*}} \upsilon (1)=0\), we get that
From (7), we have
and hence, by the above notations displayed in (5), we get
Inserting the obtained value for \(c_{1}\) into (8), we reach
and by (5) this turns into
and so again by (5) it becomes
On the other hand, putting the value of \(c_{2}\) in (9), we try to find \(c_{1}\) as
which implies
and so
Finally, in view of notations (5), we have
At last, we put values of \(c_{0}\), \(c_{1}\), \(c_{2}\) computed by the above procedures in (6), and we reach
This completes our proof about the structure of solutions for BVP (3). □
Inspired by the previous lemma, we now assume an operator \(\mathfrak{T}: \mathcal{M}\to \mathcal{M}\) defined by
It is to be noted that \(\upsilon _{0}\) is regarded as a solution for supposed BVP (1) iff \(\upsilon _{0}\) is a fixed point for the newly-defined map \(\mathfrak{T}\). Set:
We are at this moment ready to express and verify the first theorem pertinent to the existence conditions of solutions for BVP (1).
Theorem 3.2
Let \(\psi \in \Pi \) and \(\chi ^{*} :\mathbb{R}^{2}\times \mathbb{R}^{2}\rightarrow \mathbb{R}\) be a map along with a continuous map \(\Phi ^{*}:\mathbb{J} \times \mathcal{M}\times \mathcal{M} \rightarrow \mathcal{M}\). Suppose that:
-
(i)
for all \(\upsilon _{1}, \upsilon _{1}', \upsilon _{2}, \upsilon _{2}'\in \mathcal{M}\) with \(\chi ^{*}((\upsilon _{1}(z), \upsilon _{2}(z)),(\upsilon _{1}'(z), \upsilon _{2}'(z)))\geq 0\) and \(\lambda ^{*}=\frac{1}{\Omega _{1}^{*}+\Omega _{2}^{*}}\), we have
$$ \bigl\vert \Phi ^{*}\bigl(z,\upsilon _{1}(z), \upsilon _{2}(z)\bigr)-\Phi ^{*}\bigl(z, \upsilon _{1}'(z), \upsilon _{2}'(z) \bigr) \bigr\vert \leq \lambda ^{*} \psi \bigl( \bigl\vert \upsilon _{1}-\upsilon _{1}' \bigr\vert + \bigl\vert \upsilon _{2}-\upsilon _{2}' \bigr\vert \bigr); $$ -
(ii)
there exists \(\upsilon _{0}\in \mathcal{M}\) such that, for all \(z\in \mathbb{J}\),
$$ \chi ^{*}\bigl(\bigl(\upsilon _{0}(z),{}^{\mathcal{C}} \mathfrak{D}_{0}^{1} \upsilon _{0}(z) \bigr),\bigl(\mathfrak{T}\upsilon _{0}(z), {}^{\mathcal{C}} \mathfrak{D}_{0}^{1}\mathfrak{T}\upsilon _{0}(z)\bigr)\bigr)\geq 0, $$and also
$$ \chi ^{*}\bigl(\bigl(\upsilon _{1}(z),{}^{\mathcal{C}} \mathfrak{D}_{0}^{1} \upsilon _{1}(z) \bigr),\bigl(\upsilon _{2}(z), {}^{\mathcal{C}} \mathfrak{D}_{0}^{1} \upsilon _{2}(z)\bigr) \bigr)\geq 0 $$implies
$$ \chi ^{*}\bigl(\bigl(\mathfrak{T}\upsilon _{1}(z),{}^{\mathcal{C}} \mathfrak{D}_{0}^{1} \mathfrak{T}\upsilon _{1}(z)\bigr),\bigl(\mathfrak{T}\upsilon _{2}(z), {}^{ \mathcal{C}}\mathfrak{D}_{0}^{1}\mathfrak{T} \upsilon _{2}(z)\bigr)\bigr)\geq 0 $$for all \(z\in \mathbb{J} \) and \(\upsilon _{1},\upsilon _{2}\in \mathcal{M}\);
-
(iii)
for an arbitrary sequence \(\{\upsilon _{n}\}_{n\geq 1} \subseteq \mathcal{M}\) with \(\upsilon _{n}\rightarrow \upsilon \) and
$$ \chi ^{*}\bigl(\bigl(\upsilon _{n}(z),{}^{\mathcal{C}} \mathfrak{D}_{0}^{1} \upsilon _{n}(z) \bigr),\bigl(\upsilon _{n+1}(z), {}^{\mathcal{C}} \mathfrak{D}_{0}^{1} \mathfrak{T}\upsilon _{n+1}(z)\bigr)\bigr)\geq 0 $$for any \(n\in \mathbb{N}\) and \(z\in \mathbb{J}\), the following inequality holds:
$$ \chi ^{*}\bigl(\bigl(\upsilon _{n}(z),{}^{\mathcal{C}} \mathfrak{D}_{0}^{1} \upsilon _{n}(z) \bigr),\bigl(\upsilon (z), {}^{\mathcal{C}}\mathfrak{D}_{0}^{1} \mathfrak{T}\upsilon (z)\bigr)\bigr)\geq 0. $$
Then the given BVP (1) has at least one solution.
Proof
Let \(\upsilon _{1},\upsilon _{2}\in \mathcal{M}\) subject to \(\chi ^{*}((\upsilon _{1}(z),{}^{\mathcal{C}}\mathfrak{D}_{0}^{1} \upsilon _{1}(z)),(\upsilon _{2}(z), {}^{\mathcal{C}}\mathfrak{D}_{0}^{1} \upsilon _{2}(z)))\geq 0\) for every \(z\in \mathbb{J}\). In that case, one can estimate
Similarly, we have
Hence, \(|\mathfrak{T}\upsilon _{1}(z)-\mathfrak{T}\upsilon _{2}(z)|\leq ( \Omega _{1}^{*}+\Omega _{2}^{*})\lambda ^{*}\psi (\|\upsilon _{1}- \upsilon _{2}\|)= \psi (\upsilon _{1}-\upsilon _{2})\). We now build α on \(\mathcal{M}\times \mathcal{M}\) by
for all \(\upsilon _{1},\upsilon _{2}\in \mathcal{M}\). Then we have \(\alpha (\upsilon _{1}, \upsilon _{2})d(\mathfrak{T}\upsilon _{1}, \mathfrak{T}\upsilon _{2}) \leq \psi (d(\upsilon _{1}, \upsilon _{2}))\) for all \(\upsilon _{1}, \upsilon _{2} \in \mathcal{M}\). From this, we see that \(\mathfrak{T}\) is an \(\alpha -\psi \)-contraction. Moreover, one can easily observe that it is α-admissible and \(\alpha (\upsilon _{0}, \mathfrak{T}\upsilon _{0}) \geq 1\) by the definition of \(\chi ^{*}\).
Now, in this situation, for all n, we suppose that \(\{\upsilon _{n}\}\) is a sequence which belongs to \(\mathcal{M}\) with \(\upsilon _{n}\rightarrow \upsilon \) and \(\alpha (\upsilon _{n}, \upsilon _{n+1}) \geq 1\). In view of the structure of α,
Thus, by the hypothesis, we get
It validates that \(\alpha (\upsilon _{n}, \upsilon ) \geq 1\). Therefore, Banach space \(\mathcal{M}\) has the property (B). From Theorem 2.12, we find that \(\mathfrak{T}\) has \(\upsilon ^{*}\in \mathcal{M}\) as a fixed point, and in consequence, the supposed BVP (1) contains a solution. The argument is finished. □
By following the argument, we focus on our intention to find other existence requirements of solutions for BVP (1) by means of another tool based on the fixed point.
Theorem 3.3
Consider a continuous function \(\Phi ^{*}: \mathbb{J}\times \mathcal{M}\times \mathcal{M} \rightarrow \mathcal{M}\). Suppose that:
-
(iv)
A continuous function ξ is formulated on the closed interval \(\mathbb{J}\) such that
$$ \bigl\vert \Phi ^{*}(z,\upsilon _{1},\upsilon _{2})-\Phi ^{*}\bigl(z,\upsilon _{1}', \upsilon _{2}'\bigr) \bigr\vert \leq \xi (z) \Biggl( \sum_{k=1}^{2} \bigl\vert \upsilon _{k}- \upsilon _{k}' \bigr\vert \Biggr) $$for all \(z\in \mathbb{J}\) and \(\upsilon _{1}, \upsilon _{2}, \upsilon _{1}', \upsilon _{2}'\in \mathcal{M}\);
-
(v)
There are a continuous function \(\eta ^{*}: \mathbb{J} \rightarrow \mathbb{R}^{+}\) and a nondecreasing function \(\psi : \mathbb{R}^{+} \rightarrow \mathbb{R}^{+}\) such that
$$ \bigl\vert \Phi ^{*}(z, \upsilon _{1}, \upsilon _{2}) \bigr\vert \leq \eta ^{*}(z) \psi \Biggl(\sum _{k=1}^{2} \vert \upsilon _{k} \vert \Biggr) $$for all \(z\in \mathbb{J}\) and \(\upsilon _{1}, \upsilon _{2}\in \mathcal{M}\).
Then BVP (1) has at least one solution whenever
by terms of constants \(\Omega _{3}^{*}\) and \(\Omega _{4}^{*}\) given by (10).
Proof
Let \(\|\eta ^{*}\|=\sup_{z\in \mathbb{J}}|\eta ^{*}(z)|\) and put \(m^{*}=\sup_{\upsilon \in \mathcal{M}} \psi (\|\upsilon \|)\), and assume that there is \(\epsilon > 0 \) such that
where \(\Omega _{i}^{*}\)s are given in (10). We define a set \(\mathcal{A}_{\epsilon }=\{\upsilon \in \mathcal{M}:\|\upsilon \|\leq \epsilon \}\), and it is easy to verify that \(\mathcal{A}_{\epsilon }\neq \emptyset \) belongs to \(\mathcal{P}_{CL}(\mathcal{M})\), \(\mathcal{P}_{CV}(\mathcal{M})\), and \(\mathcal{P}_{BD}(\mathcal{M})\) simultaneously. Now we define two fractional operators \(\mathfrak{T}^{1}\) and \(\mathfrak{T}^{2}\) on a set \(\mathcal{A}_{\epsilon }\) by
and
For all \(z\in \mathbb{J}\) and for \(\upsilon _{1}, \upsilon _{2}\in \mathcal{A}_{\epsilon }\), we have
Also
Hence \(\Vert \mathfrak{T}^{1}\upsilon _{1}(z)+\mathfrak{T}^{2}\upsilon _{2}(z) \Vert \leq \epsilon \), which implies that \(\mathfrak{T}^{1}\upsilon _{1}(z)+\mathfrak{T}^{2}\upsilon _{2}(z) \in \mathcal{A}_{\epsilon }\). On the other hand, since \(\Phi ^{*}\) is continuous, this ensures that \(\mathfrak{T}^{1}\) will be continuous. Moreover, along with these, we compute
and
for all \(\upsilon \in \mathcal{A}_{\epsilon }\). Hence,
which guarantees that \(\mathfrak{T}^{1}\) is uniformly bounded on \(\mathcal{A}_{\epsilon }\). Now, in the next step, we show that the operator \(\mathfrak{T}^{1}\) is compact on \(\mathcal{A}_{\epsilon }\), for this we assume that \(z_{1},z_{2}\in \mathbb{J}\) with \(z_{2}>z_{1}\). Thus we have
So \(|\mathfrak{T}^{1}\upsilon (z_{2})-\mathfrak{T}^{1}\upsilon (z_{1})| \to 0\) when \(z_{1} \to z_{2}\). Also, in a similar manner,
which implies that \(|{}^{\mathcal{C}}\mathfrak{D}_{0}^{1}\mathfrak{T}^{1}\upsilon (z_{2})-{}^{\mathcal{C}}\mathfrak{D}_{0}^{1}\mathfrak{T}^{1}\upsilon (z_{1})| \rightarrow 0\) as \(z_{1} \to z_{2}\); hence \(\|\mathfrak{T}^{1}\upsilon (z_{2})-\mathfrak{T}^{1}\upsilon (z_{1}) \|\rightarrow 0\) as \(z_{1}\rightarrow z_{2}\). Thus \(\mathfrak{T}^{1}\) is equicontinuous and \(\mathfrak{T}^{1}\) is relatively compact on \(\mathcal{A}_{\epsilon }\). Now, by the Arzela–Ascoli theorem, it is compact. Finally, we prove that \(\mathfrak{T}^{2}\) is a contraction. For \(\upsilon _{1} , \upsilon _{2} \in \mathcal{A}_{\epsilon }\), we get
also
Therefore, we get
where \(\|\xi \|=\sup_{z\in \mathbb{J}}|\xi (z)|\). Hence, \(\|\mathfrak{T}^{2}\upsilon _{1}(z)-\mathfrak{T}^{2}\upsilon _{2}(z) \|\leq (\Omega _{3}^{*}+\Omega _{4}^{*})\|\upsilon _{1}-\upsilon _{2} \|\), which implies that
Thus, \(\mathfrak{T}^{2}\) is a contraction on \(\mathcal{A}_{\epsilon }\) with constant \(K^{*}=\Omega _{3}^{*}+\Omega _{4}^{*}<1\). So, from Theorem 2.13, BVP (1) has at least one solution and the proof is completed. □
4 Existence criteria for problem (2)
In this part, we derive the existence of solutions to the inclusion BVP (2). The function \(\upsilon \in C(\mathbb{J}, \mathcal{M})\) is called the solution of problem (2) when it satisfies the boundary conditions and there is \(\Upsilon \in L^{1}(\mathbb{J})\) such that \(\Upsilon (z)\in \mathfrak{H}(z, \upsilon (z), {}^{\mathcal{C}} \mathfrak{D}_{0}^{1}\upsilon (z))\) for almost all \(z\in \mathbb{J}\) and
for all \(z\in \mathbb{J}\). For each \(\upsilon \in \mathcal{M}\), we define the set of selections of the operator \(\mathfrak{H}\) as
Moreover, define the operator \(\mathfrak{N}:\mathcal{M}\to \mathcal{P}(\mathcal{M})\) by
where
Theorem 4.1
Let \(\mathfrak{H}: \mathbb{J} \times \mathcal{M} \times \mathcal{M} \rightarrow \mathcal{P}_{CP}(\mathcal{M})\) be a multifunction. Assume that:
-
(vi)
The multifunction \(\mathfrak{H}\) is integrable and bounded and \(\mathfrak{H}( \cdot , \upsilon _{1}, \upsilon _{2}): \mathbb{J} \rightarrow \mathcal{P}_{CP}(\mathcal{M})\) is measurable for \(\upsilon _{1}, \upsilon _{2}\in \mathcal{M}\);
-
(vii)
There exist \(\omega \in C(\mathbb{J}, [0,\infty ))\) and \(\psi \in \Pi \) such that
$$ \mathcal{H}_{d}\bigl(\mathfrak{H}(z,\upsilon _{1}, \upsilon _{2}), \mathfrak{H}(z,\acute{\upsilon _{1}}, \acute{\upsilon _{2}})\bigr)\leq \frac{\omega (z)\lambda ^{*}}{ \Vert \omega \Vert }\psi \Biggl( \sum_{k=1}^{2} \vert \upsilon _{k}-\acute{\upsilon _{k}} \vert \Biggr) $$for all \(z \in \mathbb{J}\) and \(\upsilon _{1},\upsilon _{2},\acute{\upsilon _{1}}, \acute{\upsilon _{2}}\in \mathcal{M}\), where \(\lambda ^{*}=\frac{1}{\Omega _{1}^{*}+\Omega _{2}^{*}}\);
-
(viii)
There is \(\chi ^{*}:\mathbb{R}^{2} \times \mathbb{R}^{2}\rightarrow \mathbb{R}\) such that \(\chi ^{*}((\upsilon _{1},\upsilon _{2}),(\acute{\upsilon _{1}}, \acute{\upsilon _{2}}))\geq 0\) for all \(\upsilon _{k},\acute{\upsilon _{k}} \in \mathcal{M}\) (\(k=1,2\));
-
(ix)
If \(\{\upsilon _{n}\}\) is a sequence in \(\mathcal{M}\) with \(\upsilon _{n}\rightarrow \upsilon \) and
$$ \chi ^{*}\bigl(\bigl(\upsilon _{n}(z),{}^{\mathcal{C}} \mathfrak{D}_{0}^{1} \upsilon _{n}(z) \bigr),\bigl(\upsilon _{n+1}(z), {}^{\mathcal{C}} \mathfrak{D}_{0}^{1} \upsilon _{n+1}(z)\bigr) \bigr)\geq 0 $$for all \(z\in \mathbb{J}\) and \(n\geq 1\), then there exists a subsequence \(\{\upsilon _{n_{j}}\}\) of \(\{\upsilon _{n}\}\) such that
$$ \chi ^{*}\bigl(\bigl(\upsilon _{n_{j}}(z),{}^{\mathcal{C}} \mathfrak{D}_{0}^{1} \upsilon _{n_{j}}(z) \bigr),\bigl(\upsilon (z), {}^{\mathcal{C}}\mathfrak{D}_{0}^{1} \upsilon (z)\bigr)\bigr)\geq 0 $$for \(z\in \mathbb{J}\) and \(j\geq 1\);
-
(x)
There exist \(\upsilon _{0}\in \mathcal{M}\) and \(\mathfrak{p}\in \mathfrak{N}(\upsilon _{0})\) such that
$$ \chi ^{*}\bigl(\bigl(\upsilon _{0}(z),{}^{\mathcal{C}} \mathfrak{D}_{0}^{1} \upsilon _{0}(z) \bigr),\bigl(\mathfrak{p}(z), {}^{\mathcal{C}}\mathfrak{D}_{0}^{1} \mathfrak{p}(z)\bigr)\bigr)\geq 0\quad (z\in \mathbb{J}), $$in which \(\mathfrak{N}:\mathcal{M}\rightarrow P(\mathcal{M})\) is defined by (13);
-
(xi)
For any \(\upsilon \in \mathcal{M}\) and \(\mathfrak{p}\in \mathfrak{N}(\upsilon )\) with
$$ \chi ^{*}\bigl(\bigl(\upsilon (z),{}^{\mathcal{C}} \mathfrak{D}_{0}^{1}\upsilon (z)\bigr),\bigl( \mathfrak{p}(z), {}^{\mathcal{C}}\mathfrak{D}_{0}^{1} \mathfrak{p}(z)\bigr)\bigr) \geq 0, $$there exists a member \(w^{*}\in \mathfrak{N}(\upsilon )\) such that
$$ \chi ^{*}\bigl(\bigl(\mathfrak{p}(z),{}^{\mathcal{C}} \mathfrak{D}_{0}^{1} \mathfrak{p}(z)\bigr), \bigl(w^{*}(z), {}^{\mathcal{C}}\mathfrak{D}_{0}^{1}w^{*}(z) \bigr)\bigr) \geq 0 $$for all \(z\in \mathbb{J}\).
In that case, the inclusion problem (2) has at least one solution.
Proof
Obviously, the fixed point of \(\mathfrak{N}:\mathcal{M}\rightarrow P(\mathcal{M})\) is the solution of BVP (2). Since the multi-valued map \(z\rightarrow \mathfrak{H}(z,\upsilon (z),{}^{\mathcal{C}} \mathfrak{D}_{0}^{1}\upsilon (z))\) is closed-valued and measurable for all \(\upsilon \in \mathcal{M}\), so \(\mathfrak{H}\) has a measurable selection and \(\mathfrak{S}_{\mathfrak{H},\upsilon }\) is nonempty. We have to prove that \(\mathfrak{N}(\upsilon )\) is closed in \(\mathcal{M}\) for \(\upsilon \in \mathcal{M}\). Take \(\{\upsilon _{n}\}\) in \(\mathfrak{N}(\upsilon )\) that \(\upsilon _{n} \to \upsilon \). For each n, \(\Upsilon _{n}\in \mathfrak{S}_{\mathfrak{H},\upsilon }\) is chosen such that
for all \(z\in \mathbb{J}\). Since \(\mathfrak{H}\) has compact values, we define a subsequence of \(\{\Upsilon _{n}\}\) (again by the same notation) which converges to \(\Upsilon \in L^{1}([0,1])\). Hence, \(\Upsilon \in \mathfrak{S}_{\mathfrak{H},\upsilon }\) and
for all \(z\in \mathbb{J}\), which gives \(\upsilon \in \mathfrak{N}(\upsilon )\) and \(\mathfrak{N}\) is closed-valued. As \(\mathfrak{H}\) is compact-valued, it is a simple task to affirm the boundedness of \(\mathfrak{N}(\upsilon )\) for arbitrary \(\upsilon \in \mathcal{M}\). We have to prove that \(\mathfrak{N}\) is an \(\alpha -\psi \)-contraction. For such a goal, we define
for all \(\upsilon ,\acute{\upsilon }\in X\). Let \(\upsilon ,\acute{\upsilon }\in X\) and \(\hslash _{1}^{*}\in \mathfrak{N}(\acute{\upsilon })\) and choose \(\Upsilon _{1}\in \mathfrak{S}_{\mathfrak{H},\acute{\upsilon }}\) such that
for all \(z\in \mathbb{J}\). We estimate
for all \(\upsilon ,\acute{\upsilon }\in \mathcal{M}\) with \(\chi ^{*}((\upsilon (z),{}^{\mathcal{C}}\mathfrak{D}_{0}^{1} \upsilon (z)),(\acute{\upsilon }(z),{}^{\mathcal{C}}\mathfrak{D}_{0}^{1} \acute{\upsilon }(z)))\geq 0\) for almost all \(z\in \mathbb{J}\). Thus there exists \(\pi \in \mathfrak{H}(z,\upsilon (z),{}^{\mathcal{C}}\mathfrak{D}_{0}^{1} \upsilon (z))\) such that
Now let \(\mathfrak{B}^{*}:[0,1]\rightarrow \mathcal{P}(\mathcal{M})\) be a multi-valued map as
for all \(z\in \mathbb{J}\). As \(\Upsilon _{1}\) and \(\zeta =\frac{\omega \lambda ^{*}}{\|\omega \|}\psi (|\upsilon _{1}- \acute{\upsilon _{1}}|-|{}^{\mathcal{C}}\mathfrak{D}_{0}^{1} \acute{\upsilon }- {}^{\mathcal{C}}\mathfrak{D}_{0}^{1} \acute{\upsilon }|)\) are measurable, the multi-valued function \(\mathfrak{B}^{*}(\cdot )\cap \mathfrak{H}(\cdot ,\upsilon (\cdot ),{}^{ \mathcal{C}}\mathfrak{D}_{0}^{1}\upsilon (\cdot ))\) is too. Now let \(\Upsilon _{2}\in \mathfrak{H}(z,\upsilon (z),{}^{\mathcal{C}} \mathfrak{D}_{0}^{1}\upsilon (z))\) provided
for all \(z\in \mathbb{J}\). Let us define \(\hslash _{2}^{*}\in \mathfrak{N}(z)\) by
for all \(z\in \mathbb{J}\). Let \(\sup_{z\in \mathbb{J}}|\omega (z)|=\|\omega \|\). Then
Also we have
for all \(z\in \mathbb{J}\). Hence
Thus \(\alpha (\upsilon ,\acute{\upsilon })\mathcal{H}_{d}(\mathfrak{N}( \upsilon ),\mathfrak{N}(\acute{\upsilon }))\leq \psi (\|\upsilon - \acute{\upsilon }\|)\) holds for all \(\upsilon ,\acute{\upsilon }\in \mathcal{M}\), which implies that \(\mathfrak{N}\) is an \(\alpha -\psi \)-contraction. Now, let \(\upsilon \in \mathcal{M}\) and \(\acute{\upsilon }\in \mathfrak{N}(\upsilon )\) be two functions such that \(\alpha (\upsilon ,\acute{\upsilon })\geq 1\). In this case, \(\chi ^{*}((\upsilon (z),{}^{\mathcal{C}}\mathfrak{D}_{0}^{1} \upsilon (z)),(\acute{\upsilon }(z),{}^{\mathcal{C}}\mathfrak{D}_{0}^{1} \acute{\upsilon }(z)))\geq 0\); so, \(\pi \in \mathfrak{N}(\acute{\upsilon })\) exists such that \(\chi ^{*}((\acute{\upsilon }(z),{}^{\mathcal{C}}\mathfrak{D}_{0}^{1} \acute{\upsilon }(z)),(\pi (z),{}^{\mathcal{C}}\mathfrak{D}_{0}^{1} \pi (z)))\geq 0\). It follows from this that \(\alpha (\acute{\upsilon },\pi )\geq 1\), which means that the operator \(\mathfrak{N}\) is α-admissible. Now, suppose that \(\upsilon _{0}\in \mathcal{M}\) and \(\acute{\upsilon }\in \mathfrak{N}(\upsilon _{0})\) are such that \(\chi ^{*}((\upsilon _{0}(z),{}^{\mathcal{C}}\mathfrak{D}_{0}^{1} \upsilon _{0}(z)),(\acute{\upsilon }(z),{}^{\mathcal{C}}\mathfrak{D}_{0}^{1} \acute{\upsilon }(z)))\geq 0\) for any \(z\in \mathbb{J}\). Subsequently, we have \(\alpha (\upsilon _{0},\acute{\upsilon })\geq 1\). Consider \(\{\upsilon _{n}\} \subseteq \mathcal{M}\) coupled with \(\upsilon _{n}\rightarrow \upsilon \) and \(\alpha (\upsilon _{n},\upsilon _{n+1})\geq 1\). Then we get
By using hypothesis (ix), there is a subsequence \(\{\upsilon _{n_{j}}\}\) of \(\{\upsilon _{n}\}\) such that
Thus \(\alpha (\upsilon _{n_{j}},\upsilon )\geq 1\) (∀j); that is, \(\mathcal{M}\) has the property \(C_{\alpha }\). Theorem 2.14 guarantees that \(\mathfrak{N}\) has a fixed point which is the solution of the inclusion BVP (2). □
Theorem 4.2
Consider the multifunction \(\mathfrak{H}: \mathbb{J}\times \mathcal{M}\times \mathcal{M} \rightarrow \mathcal{P}(\mathcal{M})\). Assume that:
-
(xii)
\(\psi : \mathbb{R}_{\geq 0}\rightarrow \mathbb{R}_{\geq 0}\) is u.s.c nondecreasing map with \(\liminf_{z\rightarrow \infty }(z-\psi (z))>0\), \(\psi (z)< z\) for all \(z>0\);
-
(xiii)
The operator \(\mathfrak{H}: \mathbb{J} \times \mathcal{M}\times \mathcal{M} \rightarrow \mathcal{P}_{CP}(\mathcal{M})\) is integrable bounded and \(\mathfrak{H}(\cdot ,\upsilon _{1}'\upsilon _{2}'): \mathbb{J} \rightarrow \mathcal{P}_{CP}(\mathcal{M})\) is measurable for all \(\upsilon _{1},\upsilon _{2}\in \mathcal{M}\);
-
(xiv)
There is \(\omega \in C(\mathbb{J},[0,\infty ))\) with
$$ \mathcal{H}_{d}\bigl(\mathfrak{H}(z,\upsilon _{1}, \upsilon _{2})- \mathfrak{H}(z,\acute{\upsilon }_{1}, \acute{\upsilon }_{2})\bigr)\leq \omega (z)\lambda ^{*} \psi \Biggl( \sum_{k=1}^{2} \vert \upsilon _{k}- \acute{\upsilon }_{k} \vert \Biggr) $$for all \(\upsilon _{k},\acute{\upsilon }_{k}\in \mathcal{M}\) (\(k=1,2\)), where \(\lambda ^{*} = \frac{1}{\Omega _{1}^{*}+\Omega _{2}^{*}} \);
-
(xv)
\(\mathfrak{N}\) has the (AEP)-property.
Then the inclusion BVP (2) has a solution.
Proof
We have to prove that \(\mathfrak{N}:\mathcal{M}\rightarrow \mathcal{P}(\mathcal{M})\) includes endpoints. Firstly, we must prove that \(\mathfrak{N}(\upsilon )\) is closed for every \(\upsilon \in \mathcal{M}\). Since the mapping \(z\rightarrow \mathfrak{H}(z,\upsilon (z),{}^{\mathcal{C}} \mathfrak{D}_{0}^{1}\upsilon (z))\) is closed-valued and measurable for \(\upsilon \in \mathcal{M}\), thus it has a measurable selection and \(\mathfrak{S}^{*}_{\mathfrak{H},\upsilon }\neq \emptyset \). By applying the same deduction done in the proof of Theorem 4.1, one may simply verify that \(\mathfrak{N}(\upsilon )\) is closed. Also, \(\mathfrak{N}(\upsilon )\) is bounded because of the compactness of \(\mathfrak{H}\). Finally, it is simple to prove that \(\mathcal{H}_{d}(\mathfrak{N}(\upsilon ),\mathfrak{N}(\pi ))\leq \psi (\|\upsilon -\pi \|)\) holds. Suppose that \(\upsilon ,\pi \in \mathcal{M}\) and \(\hslash _{1}^{*}\in \mathfrak{N}(\pi )\). Select \(\Upsilon _{1}\in \mathfrak{S}_{\mathfrak{H},\pi }\) such that
for all \(z\in \mathbb{J}\). As
for all \(z\in \mathbb{J}\), there exists \(\varpi ^{*}\in \mathfrak{H}(z,\upsilon (z),{}^{\mathcal{C}} \mathfrak{D}_{0}^{1}\upsilon (z))\) such that
for all \(z\in \mathbb{J}\). Consider the multi-valued map \(\mathfrak{O}^{*}: \mathbb{J} \to \mathcal{P}(\mathcal{M})\) defined by
By the measurability of \(\Upsilon _{1}\) and \(\varphi ^{*} = \omega \lambda ^{*}\psi (|\upsilon -\pi |-|{}^{ \mathcal{C}}\mathfrak{D}_{0}^{1}\upsilon -{}^{\mathcal{C}} \mathfrak{D}_{0}^{1}\pi |)\), it is obvious that the multifunction \(\mathfrak{O}^{*}(\cdot )\cap \mathfrak{H}(\cdot ,\upsilon (\cdot ),{}^{ \mathcal{C}}\mathfrak{D}_{0}^{1}\upsilon (\cdot ))\) will be also measurable. Now we take \(\Upsilon _{2}\in \mathfrak{H}(z,\upsilon (z),{}^{\mathcal{C}} \mathfrak{D}_{0}^{1}\upsilon (z))\) such that
for all \(z\in \mathbb{J}\). Select \(\hslash _{2}^{*}\in \mathfrak{N}(\upsilon )\) such that
for all \(z\in \mathbb{J}\). By the same argument used in Theorem 4.1, we get
Hence, \(\mathcal{H}_{d}(\mathfrak{N}(\upsilon ),\mathfrak{N}(\pi ))\leq \psi (\|\upsilon - \pi \|)\) for all \(\upsilon , \pi \in \mathcal{M}\). By using hypothesis (xv), one can easily find that \(\mathfrak{N}\) has the (AEP)-property. By Theorem 2.15, there exists \(\upsilon ^{*}\in \mathcal{M}\) such that \(\mathfrak{N}(\upsilon ^{*})=\{\upsilon ^{*}\}\). This implies that \(\upsilon ^{*}\) satisfies the given problem (2) and the proof is completed. □
5 Examples
Here, we provide two simulative examples to review our findings by a numerical view.
Example 5.1
Consider the boundary value problem in the multi-term form as follows:
where the numerical data \(\sigma ^{*}= 0.2\), \(\delta ^{*}=1.5\), \(a_{1}=2\), \(a_{2}=3\), \(a_{3}=4\), \(a_{4}=5\), \(\alpha _{1}^{*}=2\), and \(\theta ^{*}=2\) are chosen. Here, \({}^{\mathcal{C}}\mathfrak{D}_{0}^{(\cdot )}\) and \({{}^{\mathcal{RL}}}\mathfrak{I}_{0}^{(\cdot )}\) denote derivative and integral operators of the Caputo and Riemann–Liouville types. Consider the continuous function \(\Phi ^{*}: \mathbb{J} \times \mathbb{R}^{2}\rightarrow \mathbb{R}\) as
For \(s_{1},s_{2},r_{1},r_{2}\in \mathbb{R}\), we have
Put \(\xi (z)=\frac{z}{8+ 16z}\) for all \(z\in \mathbb{J}\), then \(\|\xi \|= \frac{1}{8} = 0.125\). Also, consider the continuous and nondecreasing function \(\psi : \mathbb{R}^{+} \rightarrow \mathbb{R}^{+}\) defined by \(\psi (\upsilon ) = \upsilon \) for \(\upsilon \in \mathbb{R}^{+}\). Then
It is clear that \(\eta ^{*} : \mathbb{J} \rightarrow \mathbb{R}^{+}\) defined by \(\eta ^{*} =\frac{z}{8+ 16z}\) is a continuous function with \(\|\eta ^{*}\|=0.125\). On the other hand, we get \(\nabla _{1}=-1.5\), \(\Delta _{1}=5.5956\), \(\nabla _{2}=-0.8935\), \(\nabla _{3} =-0.2233\), \(\nabla _{4}=1.5950\), \(\nabla _{5}=0.7559\), \(\nabla _{6}=-0.7441\), \(\Omega _{1}^{*}=0.7492\), \(\Omega _{2}^{*}=1.1119\), \(\Omega _{3}^{*}=0.0678\), \(\Omega _{4}^{*}=0.0822\). Since \(K^{*}= 0.1500<1\), by using Theorem 3.3, boundary value problem (14) has at least one solution.
Example 5.2
Consider the inclusion BVP in the multi-term form as follows:
where the numerical data \(\sigma ^{*}= 0.7\), \(\delta ^{*}=1.7\), \(\alpha _{1}^{*}=3\), \(\theta ^{*}=4\) and \(a_{1}=11\), \(a_{2}=12\), \(a_{3}=13\), \(a_{4}=14\) are chosen. In view of the above data, we obtain \(\nabla _{1}=-1.090\), \(\Delta _{1}=25.7554\), \(\nabla _{2}=-0.5435\), \(\nabla _{3} =0.0049\), \(\nabla _{4}=1.0155\), \(\nabla _{5}=-0.00119\), \(\nabla _{6}=-1.1019\), \(\Omega _{1}^{*}=0.4485\), \(\Omega _{2}^{*}=0.5798\), \(\Omega _{3}^{*}=0.0182\), \(\Omega _{4}^{*}=0.0182\). Here, \({}^{\mathcal{C}}\mathfrak{D}_{0}^{(\cdot )}\) and \({{}^{\mathcal{RL}}}\mathfrak{I}_{0}^{(\cdot )}\) denote derivative and integral operators of the Caputo and Riemann–Liouville types. Let \(\mathfrak{H}:\mathbb{J} \times \mathbb{R}^{2}\rightarrow \mathbb{R}\) be a multifunction defined by
For \(s_{1},s_{2},r_{1},r_{2}\in \mathbb{R}\), we have
where \(\Omega _{1}^{*} + \Omega _{2}^{*} = 1.0283\) and \(\frac{1}{\Omega _{1}^{*} + \Omega _{2}^{*}} = 0.97247 \). Choose the nonnegative function \(\omega \in C(\mathbb{J}, [0,\infty ))\) defined by \(\omega (z) =\frac{z}{20}\) for all \(z\in \mathbb{J}\). Then \(\Vert \omega \Vert = \frac{1}{20}=0.05\). Also, we consider the nonnegative and nondecreasing u.s.c map \(\psi : \mathbb{R}_{\geq 0}\rightarrow \mathbb{R}_{\geq 0}\) defined by \(\psi (z) = \frac{z}{3}\) for almost all \(z > 0\). Note that \(\lim_{z\rightarrow \infty } \inf (z-\psi (z)) > 0\) holds with \(\psi (z) < z\) (\(\forall z > 0\)). At last, consider \(\mathfrak{N}:\mathcal{M}\to \mathcal{P}(\mathcal{M})\) by
where we have
Since operator \(\mathfrak{N}\) has the (AEP)-property, by using Theorem 4.2, there exists at least one solution for the given BVP (15).
6 Conclusion
In the current research, we discussed some new conditions ensuring the existence of solution for given two multi-term BVPs in two different equation and inclusion versions. Indeed, we defined some operators based on the equivalent integral equation which belonged to a special category of α-admissible and α-ψ-contractions. Also, we investigated the (AEP)-property for such operators. At last, in two separate examples, the numerical simulation of given BVPs was done. As a future and next project, one can apply these techniques for the generalized BVPs having multi-strip, multi-point, multi-order integral conditions simultaneously.
Availability of data and materials
Data sharing not applicable to this article as no datasets were generated or analyzed during the current study.
References
Adjabi, Y., Samei, M.E., Matar, M.M., Alzabut, J.: Langevin differential equation in frame of ordinary and Hadamard fractional derivatives under three point boundary conditions. AIMS Math. 6(3), 2796–2843 (2021). https://doi.org/10.3934/math.2021171
Baleanu, D., Etemad, S., Rezapour, S.: A hybrid Caputo fractional modeling for thermostat with hybrid boundary value conditions. Bound. Value Probl. 2020, 64 (2020). https://doi.org/10.1186/s13661-020-01361-0
Baleanu, D., Jajarmi, A., Mohammadi, H., Rezapour, S.: Analysis of the human liver model with Caputo–Fabrizio fractional derivative. Chaos Solitons Fractals 134, 109705 (2020). https://doi.org/10.1016/j.chaos.2020.109705
Bonilla, B., Rivero, M., Rodriguez-Germa, L., Trujillo, J.J.: On Hadamard fractional integro-differential boundary value problems. Appl. Math. Comput. 187(1), 79–88 (2007). https://doi.org/10.1016/j.amc.2006.08.105
Khan, S.A., Shah, K., Zaman, G., Jarad, F.: Existence theory and numerical solutions to smoking model under Caputo–Fabrizio fractional derivative. Chaos, Interdiscip. J. Nonlinear Sci. 29(3), 013128 (2019). https://doi.org/10.1063/1.5079644
Pratap, A., Raja, R., Alzabut, J., Cao, J., Rajchakit, G., Huang, C.: Mittag-Leffler stability and adaptive impulsive synchronization of fractional order neural networks in quaternion field. Math. Methods Appl. Sci. 43(10), 6223–6253 (2020). https://doi.org/10.1002/mma.6367
Pratap, A., Raja, R., Alzabut, J., Dianavinnarasi, J., Cao, J., Rajchakit, G.: Finite-time Mittag-Leffler stability of fractional order quaternion-valued memristive neural networks with impulses. Neural Process. Lett. 51, 1485–1526 (2020). https://doi.org/10.1007/s11063-019-10154-1
Thabet, S.T.M., Etemad, S., Rezapour, S.: On a new structure of the pantograph inclusion problem in the Caputo conformable setting. Bound. Value Probl. 2020, 171 (2020). https://doi.org/10.1186/s13661-020-01468-4
Agarwal, R.P., Meehan, M., O’Regan, D.: Fixed Point Theory and Applications. Cambridge University Press, Cambridge (2001)
Ahmad, B., Alghamdi, N., Alsaedi, A., Ntouyas, S.K.: On multi-term fractional differential equations with multi-point boundary conditions. Eur. Phys. J. Spec. Top. 226, 3369–3390 (2017). https://doi.org/10.1140/epjst/e2018-00009-3
Ahmad, B., Matar, M.M., Ntouyas, S.K.: On general fractional differential inclusions with nonlocal integral boundary conditions. Differ. Equ. Dyn. Syst. 28, 241–254 (2020). https://doi.org/10.1007/s12591-016-0319-5
Ben-Chikh, S., Amara, A., Etemad, S., Rezapour, S.: On Hyers–Ulam stability of a multi-order boundary value problems via Riemann–Liouville derivatives and integrals. Adv. Differ. Equ. 2020, 547 (2020). https://doi.org/10.1186/s13662-020-03012-1
Berhail, A., Tabouache, N., Matar, M.M., Alzabut, J.: On nonlocal integral and derivative boundary value problem of nonlinear Hadamard–Langevin equation with three different fractional orders. Bol. Soc. Mat. Mexicana 6, 303–318 (2020). https://doi.org/10.1007/s40590-019-00257-z
Derbazi, C., Baitiche, Z., Benchohra, M., Cabada, A.: Initial value problem for nonlinear fractional differential equations with ψ-Caputo derivative via monotone iterative technique. Axioms 9(2), 57 (2020). https://doi.org/10.3390/axioms9020057
Etemad, S., Ntouyas, S.K., Tariboon, J.: Existence results for three-point boundary value problems for nonlinear fractional differential equations. J. Nonlinear Sci. Appl. 9(5), 2105–2116 (2016). https://doi.org/10.22436/jnsa.009.05.16
Etemad, S., Rezapour, S., Samei, M.E.: On a fractional Caputo–Hadamard inclusion problem with sum boundary value conditions by using approximate endpoint property. Math. Methods Appl. Sci. 43(17), 9719–9734 (2020). https://doi.org/10.1002/mma.6644
Hussain, A., Kanwal, T.: Existence and uniqueness for a neutral differential problem with unbounded delay via fixed point results. Trans. A. Razmadze Math. Inst. 172(3), 481–490 (2018). https://doi.org/10.1016/j.trmi.2018.08.006
Osler, T.J.: Leibniz rule for fractional derivatives generalized and an application to infinite series. SIAM J. Appl. Math. 18(3), 658–674 (1970). www.jstor.org/stable/2099520
Hussain, A., Yaqoob, S., Abdeljawad, T., Ur Rehman, H.: Multivalued weakly Picard operators via simulation functions with application to functional equations. AIMS Math. 6(3), 2078–2093 (2021). https://doi.org/10.3934/math.2021127
Matar, M.M., Lubbad, A.A., Alzabut, J.: On p-Laplacian boundary value problems involving Caputo–Katugampola fractional derivatives. Math. Methods Appl. Sci. (2020). https://doi.org/10.1002/mma.6534
Seemab, A., Ur Rehmanand, M., Alzabut, J., Hamdi, A.: On the existence of positive solutions for generalized fractional boundary value problems. Bound. Value Probl. 2019, 186 (2019). https://doi.org/10.1186/s13661-019-01300-8
Tariboon, J., Cuntavepanit, A., Ntouyas, S.K., Nithiarayaphaks, W.: Separated boundary value problems of sequential Caputo and Hadamard fractional differential equations. J. Funct. Spaces 2018, Article ID 6974046 (2018). https://doi.org/10.1155/2018/6974046
Mahmudov, N.I., Al-Khateeb, A.: Existence and Ulam–Hyers stability of coupled sequential fractional differential equations with integral boundary conditions. J. Inequal. Appl. 2019, 165 (2019). https://doi.org/10.1186/s13660-019-2115-6
Mohammadi, H., Etemad, S., Rezapour, S., Baleanu, D.: Two sequential fractional hybrid differential inclusions. Adv. Differ. Equ. 2020, 385 (2020). https://doi.org/10.1186/s13662-020-02850-3
Podlubny, I.: Fractional Differential Equations. Academic Press, San Diego (1999)
Samko, S.G., Kilbas, A.A., Marichev, O.I.: Fractional Integrals and Derivatives: Theory and Applications. Gordon & Breach, Philadelphia (1993)
Kilbas, A.A., Srivastava, H.M., Trujillo, J.J.: Theory and Applications of Fractional Differential Equations. Elsevier, Amsterdam (2006)
Amini-Harandi, A.: Endpoints of set-valued contractions in metric spaces. Nonlinear Anal., Theory Methods Appl. 72(1), 132–134 (2010). https://doi.org/10.1016/j.na.2009.06.074
Samet, B., Vetro, C., Vetro, P.: Fixed point theorems for α-ψ-contractive type mappings. Nonlinear Anal., Theory Methods Appl. 75(4), 2154–2165 (2018). https://doi.org/10.1016/j.na.2011.10.014
Mohammadi, B., Rezapour, S., Shahzad, N.: Some results on fixed points of α-ψ-Ciric generalized multifunctions. Fixed Point Theory Appl. 2013, 24 (2013). https://doi.org/10.1186/1687-1812-2013-24
Smart, D.R.: Fixed Point Theorems. Cambridge University Press, Cambridge (1980)
Acknowledgements
The fourth and fifth authors were supported by Azarbaijan Shahid Madani University. The authors express their gratitude to dear unknown referees for their helpful suggestions which improved the final version of this paper.
Funding
Not applicable.
Author information
Authors and Affiliations
Contributions
The authors declare that the study was realized in collaboration with equal responsibility. All authors read and approved the final manuscript.
Corresponding author
Ethics declarations
Ethics approval and consent to participate
Not applicable.
Competing interests
The authors declare that they have no competing interests.
Consent for publication
Not applicable.
Rights and permissions
Open Access This article is licensed under a Creative Commons Attribution 4.0 International License, which permits use, sharing, adaptation, distribution and reproduction in any medium or format, as long as you give appropriate credit to the original author(s) and the source, provide a link to the Creative Commons licence, and indicate if changes were made. The images or other third party material in this article are included in the article’s Creative Commons licence, unless indicated otherwise in a credit line to the material. If material is not included in the article’s Creative Commons licence and your intended use is not permitted by statutory regulation or exceeds the permitted use, you will need to obtain permission directly from the copyright holder. To view a copy of this licence, visit http://creativecommons.org/licenses/by/4.0/.
About this article
Cite this article
Baleanu, D., Iqbal, M.Q., Hussain, A. et al. On solutions of fractional multi-term sequential problems via some special categories of functions and (AEP)-property. Adv Differ Equ 2021, 197 (2021). https://doi.org/10.1186/s13662-021-03356-2
Received:
Accepted:
Published:
DOI: https://doi.org/10.1186/s13662-021-03356-2