Abstract
Background
Chagas disease (caused by Trypanosoma cruzi) is the most important neglected tropical disease (NTD) in Latin America, infecting an estimated 5.7 million people in the 21 countries where it is endemic. It is one of the NTDs targeted for control and elimination by the 2020 London Declaration goals, with the first goal being to interrupt intra-domiciliary vector-borne T. cruzi transmission. A key question in domestic T. cruzi transmission is the role that synanthropic animals play in T. cruzi transmission to humans. Here, we ask, (1) do synanthropic animals need to be targeted in Chagas disease prevention policies?, and (2) how does the presence of animals affect the efficacy of vector control?
Methods
We developed a simple mathematical model to simulate domestic vector-borne T. cruzi transmission and to specifically examine the interaction between the presence of synanthropic animals and effects of vector control. We used the model to explore how the interactions between triatomine bugs, humans and animals impact the number and proportion of T. cruzi-infected bugs and humans. We then examined how T. cruzi dynamics change when control measures targeting vector abundance are introduced into the system.
Results
We found that the presence of synanthropic animals slows the speed of T. cruzi transmission to humans, and increases the sensitivity of T. cruzi transmission dynamics to vector control measures at comparable triatomine carrying capacities. However, T. cruzi transmission is amplified when triatomine carrying capacity increases with the abundance of syntathoropic hosts.
Conclusions
Our results suggest that in domestic T. cruzi transmission scenarios where no vector control measures are in place, a reduction in synanthropic animals may slow T. cruzi transmission to humans, but it would not completely eliminate transmission. To reach the 2020 goal of interrupting intra-domiciliary T. cruzi transmission, it is critical to target vector populations. Additionally, where vector control measures are in place, synanthropic animals may be beneficial.
Similar content being viewed by others
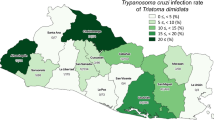
Background
Chagas disease (etiol. agent Trypanosoma cruzi), is a neglected tropical disease (NTD) endemic to the Americas, where it is vector-borne by triatomine bugs, subfamily Triatominae. An estimated 5.7 million people are infected with Chagas disease in 21 Latin American countries [1], with 10,000 Chagas-related deaths per year [2]. Thirteen percent of the Latin American population are at risk of infection [1]. Globally, Chagas disease is estimated to cost $627.5 million in healthcare costs annually and result in ~806,000 DALYs, with currently-infected individuals generating $24.7 billion in healthcare costs and 29.4 million DALYs over their lifetime [3]. With this substantial burden, Chagas disease is one of the ten NTDs targeted for control or elimination by 2020. The World Health Organization (WHO) has proposed seven milestones to combat Chagas disease by 2020, the first of which is to interrupt intra-domiciliary vector-borne transmission in Latin America [4, 5] via spraying with indoor residual insecticides (IRS) and improving housing conditions. While dwelling/housing improvement is effective in reducing T. cruzi transmission [6], it is generally expensive and time consuming. IRS can also be effective in reducing T. cruzi infection prevalence in humans [7, 8], but requires repeated spraying to avoid vector re-infestation, which can be resource intensive. To meet the 2020 Chagas control goals, these methods should be applicable and achievable across the diversity of epidemiological and ecological settings of endemic Chagas disease.
One important aspect of domestic transmission that is not included in the strategies for meeting the 2020 goal of interrupting domestic vector borne T. cruzi is the presence of synanthropic animals. These are animals that are associated with humans, whether as pets, livestock or pests. Some of these species are viable T. cruzi hosts (e.g., dogs [9, 10]), but they also include non-competent species (e.g., chickens [11]) that serve as triatomine food sources. Synanthropic animals are not currently included in the strategies for meeting the 2020 goals, yet a large body of empirical and theoretical work suggests they have a significant effect on Chagas disease dynamics ([9–17]).
Here we develop a simple model to simulate domestic vector-borne T. cruzi transmission, and more specifically, to examine the interaction between the presence of synanthropic animals and effects of vector control. We first use the model to explore how the dynamic interactions between triatomine bugs, humans and animals impact the number and proportion of T. cruzi-infected bugs and humans. As the epidemiology of Chagas disease differs widely across its range with several different vector species (within the subfamily Triatominae) and mammalian reservoir species, we have deliberately simplified our model to only consider human hosts, a single species of vector, and a homogeneous pool of reservoir hosts that vary in abundance with one division between viable and non-viable hosts. Although different synanthropic host have diverse life expectancies within and between species, as well as variable levels of T. cruzi competence, we have assumed here that these complexities can be captured by aggregating these differences into a pool of viable and non-viable hosts. The model we describe can be readily tailored to situations where the abundances of different synanthropic host species have been quantified.
To examine the effect of current policies on domestic transmission, we add in vector control methods that target vector death rates (e.g., IRS) and carrying capacity (e.g., home improvement). We aim to answer the following questions: (1) do synanthropic animals need to be targeted in Chagas disease prevention policies?, and (2) how does the presence of animals affect the efficacy of vector control?
Methods
Model structure
We assumed that the essential dynamics of Chagas disease, (defined as T. cruzi infection in humans only), could be captured using six coupled ordinary differential equations that describe the abundance of actively feeding triatomine bugs, changes in the numbers of humans with Chagas disease, and the abundance of infected synanthropic animals (of multiple, unspecified species), a proportion of which are viable T. cruzi hosts. The model was run using R software version 3.03 [18]. Ordinary differential equations were solved in R using the ‘deSolve’ package [19].
Triatomine bug dynamics
Equations 1 and 2 describe the dynamics of the triatomine bug population, which we divided into uninfected bugs, (B), and infected ‘vectors’, (V). Bugs are born at a per capita rate, r, with vectors having a modified birth rate, fr. We assume that birth rates are reduced as the total bug population approaches a finite carrying capacity, K. Vectors, V, are assumed to have fed on an infected host, and sufficient time has elapsed for the vector to become infectious at subsequent blood meals. Bugs move into the vector class through the ingestion of T. cruzi-infected blood meals from humans in one of three infection classes, (described in detail below), or infected animals (I R ), at a rate of βc x , where β represents the human-triatomine contact rate, and c x represents the probability of infection upon contact. The probabilities of infection are unique to each infection class, while the contact rate is the same, as we assume homogeneous mixing. Our current model ignores co-infection at subsequent blood meals, a complexity that has been observed to give rise to more complex dynamics in T. cruzi-infected R. prolixus [20]. We assumed that infected vectors had slightly lower fitness than uninfected vectors [20–22], represented by a scalar term f that could take values between 0–1.
We assumed that the vectors had simple dynamics driven by logistic style growth such that their abundance settles to a carrying capacity, K [23]. Initially we assume that K is independent of host abundance, but we also explore scenarios where vector carrying capacity is a function of the abundance of synanthropic animals that serve as an additional food source.
Host dynamics
We represented the total human population size by N, in which there are three stages of Chagas disease: acute infections (Ia), recently acquired and lasting 4 to 8 weeks [24]; chronic indeterminate stage infections (li), a long-term infectious period with no apparent symptoms; and chronic determinate stage infections (ld), infectious persons that develop clinically apparent symptoms (develops in 20-30 % of those in the li stage over 10 to 30 years), and can result in death. We assumed that individuals in Ia are the most infectious to triatomine bugs [25, 26] and Ii are the least infectious [27]. Humans move into the Ia class through an infective contact with vectors (V) at a rate of βc vN , after which they move from Ia to li at a rate of delta (δ) and from li to ld at a rate of sigma (σ). Individuals in ld have a Chagas disease related mortality rate of alpha (α). We assumed no superinfection of long-term patients with acute new infections. Although the time spent in each class of infection is assumed to be distributed exponentially, the net effect of allowing infections to pass through these different classes of infection is to create a more rectangular distribution of total time from initial infection to death in the final terminal infection class, as this formulation captures the main details that we are interested in. This results in three equations (3, 4, and 5) for the human population, with N-(Ia + Ii + Id) being the number of uninfected human hosts.
Equation 6 represents the non-human vertebrate species that are fed upon by vectors. We divided them into viable and non-viable T. cruzi hosts with the addition of a scalar term, pv that took values between 0–1 to represent this division; thus we ignored the relative preference of vectors for different non-human host species by including this factor. We further assumed this complex of viable reservoir species to have a common average mortality rate, μR.
Animals moved into the infected class IR through infectious contact with vectors, V at a rate of βc vR , with R - IR being the number of uninfected synanthropic animals.
Vector control and impact of control on R0
Vector control is simulated by changes in the death rates of triatomine bugs. The addition of a death rate term, D, to equations 1 and 2 results in the following equations:
We used these equations to produce an expression for the relationship between additional mortality due to triatomine vector control and the reduction in vector abundance.
Triatomines are driven to extinction when Dμb equals r, but it may also be possible to break the chain of transmission at lower levels of insecticide use. The critical level of insecticide use ‘D’ that leads to increased vector mortality Dμb and ultimately interruption of T. cruzi transmission to humans can be found by deriving an expression for the basic reproductive rate (R0) of Chagas disease using the next generation method [28].
We then used this equation to examine the relationship between R0, synanthropic animal abundance and level of insecticide use (i.e., vector mortality increase).
Data parameters and assumptions
Parameter values came from the literature (Table 1). The parameters for triatomine bug vectors were based on the species Rhodnius prolixus when possible. R. prolixus is an epidemiologically important species in northern parts of South America [29], and has average demographic rates when compared to two other key T. cruzi vector species, Triatoma infestans and Triatoma dimidiata. Parameter values for non-human host species were averaged between values available in the literature for dogs, cats, opossums, and guinea pigs, which are common synanthropic animals in many Chagas-endemic areas [14, 30, 31]. We assume frequency dependent transmission, as the vectors can only feed, defecate on, or be eaten by one host at a time, and the abundance of hosts determines how frequently this occurs. We ran the model with monthly time steps for a duration of 50 years. We assumed that all populations (humans, animals and bugs) were closed, (i.e., no immigration or emigration), and we assumed the human and animal population sizes to be constant.
Model scenarios with variation in animal presence and vector control intensity
We modeled four scenarios: human hosts only, human and animal hosts, and vector control in the presence and absence of animals. In the first scenario, humans, (N = 10, which represents a household or other small, closed population), were the only T. cruzi hosts, and we investigated the impact of the ratio of triatomine bugs to humans through changing the triatomine carrying capacity (K). Here we also examined the impact of human population size on the infection composition of humans and the triatomine bug population. In different experiments, we set K equal to 10, 50, and 100 bugs per human. As the number of triatomines in domestic settings is highly variable [32], these K values were selected to be in line with values used in other models [33, 34], and at the same time encapsulate the variation in population size reported in empirical studies [35, 36].
The second scenario further expanded the first scenario to include the animal population. We investigated the effect of animal population size and the proportion of animals that are viable T. cruzi hosts on the infection composition of humans and bugs. We first investigate this scenario with triatomine abundance independent of synanthropic animal abundance. We then examine how T. cruzi dynamics change when triatomine abundance is dependent on animal abundance by making the triatomine bug carrying capacity a linear function of synanthropic species abundance.
In the third and fourth scenario we introduced vector control into scenarios one and two, and we explored the effects of targeting triatomine carrying capacity and death rates on the infected human and bug populations. Here we used R0 to provide insight into the rates at which vectors need to be controlled in order to break the chain of transmission.
Results
Scenario 1: human hosts only
When holding all else constant, with humans (N = 10) as the only T. cruzi hosts, increasing the carrying capacity of triatomine bugs (i.e., the ratio of bugs to human hosts), increases the speed of T cruzi transmission in the system (Fig. 1). At 100 bugs per person, all 10 humans are infected with T. cruzi after 8.3 years. At 50 bugs per person, all 10 humans are infected with T. cruzi after 11.7 years. These high levels of prevalence are due to the model assumption of a closed human population. As we are mainly concerned with the interaction of vector control and synanthropic host abundance on control, we are essentially using prevalence as an index of relative risk of human infection. Prevalence begins to decrease at 10 bugs per person, where fewer than 90 % of humans have Chagas disease after 50 years, although the infections have not leveled off. Additionally, the proportion of infected bugs in the vector population remains consistent across different carrying capacities, with approximately 61 % of bugs infected at K = 1000 and K = 500, and 58 % infected at K = 100 (Fig. 1).
Number of T. cruzi-infected humans (N = 10) and triatomines at different carrying capacities with no animals in the transmission scenario. Top row: a). K = 1000, bugs and humans; b). K = 1000, just humans. Middle row: c). K = 500, bugs and humans; d). K = 500, just humans. Bottom row: e). K = 100, bugs and humans; f). K = 100, just humans. Starting conditions: B = K/2, V = 10, no infected humans
Scenario 2: human and animal hosts
The addition of synanthropic animals to the system reduces T. cruzi transmission speed and human infection prevalence if triatomine carrying capacity is not increased. As animal abundance rises, the proportion of the human population infected with T. cruzi decreases (Fig. 2b). The addition of 20 synanthropic animals (75 % viable T. cruzi hosts) reduces the speed of T. cruzi transmission to humans (N = 10), compared to when there are only human hosts. At K = 1000, all 10 humans are infected with T. cruzi after 9.3 years, one year later than without animals (8.3 years). At 50 bugs per person (K = 500), all 10 humans are infected with T. cruzi after 15.3 years (compared to 11.7 years without animal hosts). At 10 bugs per person (K = 100), there are still fewer than 8 people with Chagas disease after 50 years (one person fewer than without animals), although the number of human infections slowly continues to increase. Additionally, transmission speed is further reduced as the proportion of animals that are viable T. cruzi hosts decreases.
Number of T. cruzi-infected humans (N = 10) and triatomines by animal abundance. Top row: a). K increases linearly with animal abundance and 75 % of animals are viable T. cruzi hosts; b). K = 100 and 75 % of animals are viable hosts. Bottom row: c). K increases linearly with animal abundance and 1 % of animals are viable hosts. Simulated for 50 years and output from the final five years shown
Although the addition of synanthropic animals (n = 20) slows T. cruzi transmission to humans, it also increases the proportion of the triatomine bug population infected with T. cruzi. For K values of 1000 and 500, the population stabilizes at about 85 % of the bugs infected (75 % of animals viable), as opposed to 61 % infected without animal hosts. At K = 100, 83 % of bugs are infected, up from 58 % without animals. This effect is reduced as animal T. cruzi host competence decreases.
With triatomine bug abundance dependent on the number of the synanthropic animals in the system, T. cruzi transmission speed increases dramatically. With carrying capacity increased by 25 bugs for each animal introduced into the system and 75 % of animals viable T. cruzi hosts, the number of humans infected with T. cruzi increases slowly at all animal abundances between 5 and 200 (Fig. 2a). When most of the animals in the system are not viable T. cruzi hosts, T. cruzi transmission is slower, and the number of humans with Chagas disease begins to decline slowly as animal abundance increases (Fig. 2c and d).
Scenario 3: triatomine bug control, no animals present
The speed of T. cruzi transmission to humans (N = 10, K = 1000) is reduced with control strategies that increase the triatomine death rate by ≥50 % from the background vector mortality rate. For example, with a 75 % increase in vector death rate, the human population saturates with T. cruzi infection after 9 years, compared to 8.3 years with no intervention. Doubling the triatomine death rate, slows T. cruzi transmission even more, with saturation occurring after 11.8 years. While these increased death rates reduce the speed of T. cruzi transmission in the human population, all humans still eventually become infected. The number of humans with Chagas disease is only reduced after the triatomine bug death rate is increased by at least 7.
At a triatomine carrying capacity of 500, the dynamics are more sensitive to increases in triatomine death rate, with a 25 % death rate increase slowing saturation to 14.3 years. The number of humans with Chagas disease begins to decrease when triatomine mortality is increased by 3.25 times. At K = 100 and a 25 % increase in triatomine mortality, there are fewer than 8 people with Chagas disease after 50 years, compared with 9 people with no vector control.
Scenario 4: triatomine bug control with animals present
The addition of 20 animals (75 % viable, N = 10) to the system makes the dynamics more sensitive to changes in triatomine death rates. T. cruzi transmission to humans is slower at vector death rate increases of 1 % and higher. For example, with a vector death rate increase of 25 %, saturation of humans infected with Chagas disease occurred after 9.7 years when K = 1000 (compared to 9 years with no animals and intervention), and 15.9 years (compared to 14.3 years when K = 500). However, there is still no change in the final number of humans with Chagas disease until triatomine the death rate was increased 7-fold when K = 1000 and 3.5 fold for K = 500.
R0 and vector control in the presence of animals
R0decreases as both synanthropic animal abundance and vector mortality increase (Fig. 3). With 75 % of animals viable T. cruzi hosts and triatomine mortality doubled (N = 10, V = 500), R0 ranges from 21.42 (2 animals) to 16.14 (20 animals). With 25 % viable hosts and mortality doubled, R0 ranges from 21.21 (2 animals) to 14.01 (20 animals). R0 falls below one when the triatomine mortality rate is increased by a factor of 20 and there are at least two animals.
The relationship between R0, synanthropic animal abundance and triatomine mortality. Top: System with 10 humans. Bottom: System without humans. Run with 500 infected bugs (i.e., 'vectors') and 10 human hosts for triatomine mortality increases between 1–20 times the background rate, and 1–30 synthropic animals, of which 75 % are competent T. cruzi hosts
Without humans, R0 is further reduced (Fig. 3, bottom image). With a doubled vector mortality rate R0 is 12.04 (75 % viable animal hosts) and 6.95 (25 % viable) at all animal population sizes between 2–30. R0 drops below one when vector mortality is increased at least 16 times when 25 % of the animals are viable, and 19 times when 75 % of animals are viable.
Discussion
Our results suggests that vector control methods targeting triatomine death rates will reduce the speed of T. cruzi transmission to humans, but must be implemented at very high intensities to reduce Chagas disease prevalence and R0. The addition of synanthropic animals decreases the speed of T. cruzi transmission to humans if these hosts have no effect on triatomine abundance. However, when synanthropic animals increase triatomine carrying capacity, then higher levels of vector control are required to reduce transmission, particularly if the animals are competent T. cruzi hosts.
Synanthropic animals: a dilution effect?
Although it is not unexpected that the addition of 20 animals into the system slowed T. cruzi transmission to humans, as it diluted the ratio of bugs to hosts by two-thirds, the viable animal hosts in our model did have a higher probability of becoming infected themselves and also of infecting the triatomine bug, which is reflected in the higher proportion of infected bugs when they are added to the system. Therefore, the possibility remained that they could amplify transmission as well, even without increasing triatomine carrying capacity. Surprisingly, our simulation of R0 revealed that, at comparable carrying capacities, it is the humans that amplify transmission, probably because of their long life spans.
The ‘dilution effect’ hypothesis is defined as a decrease in infectious disease risk with an increase in species diversity [37, 38]. Although in our model we do not delineate between species beyond human and non-human, our results suggest that zooprophylaxis could occur with the addition of non-human hosts that divert T. cruzi-infected triatomine bites away from humans. Moreover, T. cruzi is considered as a parasite that responds negatively to biodiversity in undisturbed ‘wild’ systems [39], although it is unknown if this is the case in domestic transmission settings. However, arguable dilution effects have been observed in peri-domestic transmission scenarios around the Panama Canal [40]. Thus, our results support the possibility of a dilution effect, but future development of the model to include different animal species is needed and will be explored in future work.
The carrying capacity crux
Our results suggest that if measures are taken to prevent triatomine abundance from increasing with the addition of synanthropic animals, then they would not only be beneficial, but it could be possible to keep the T. cruzi R0 below 1, even without driving the triatomine population to zero. However, this is not an easy task, as synanthropic animals in domestic and peri-domestic transmission scenarios lead to an increased blood (i.e., food) supply for triatomine bugs. As obligate blood feeders, the number of eggs laid by a female triatomine is strongly correlated with the amount of blood consumed [41], so an increase in blood availability generally leads to an increased carrying capacity if sufficient triatomine habitat is available, (illustrated in the iteration of our model with triatomine density dependent on animal abundance). Therefore, triatomine carrying capacity must be reduced, or at least prevented from increasing in the presence of synanthropic animals. This is currently done with varying levels of success through housing improvements that include replacing roofing and wall materials [42], to reduce the dark and hidden microspaces preferred by domiciliary triatomines [43]. Another potential area of housing improvement is targeting abiotic factors in triatomine microhabitats such as climate [44], light and substrate [45], factors to which triatomine bugs are very sensitive.
Policy implications and the 2020 goals
Our results have several policy implications. First, as stated above, to impact the magnitude of T. cruzi spread, prevention and control measures must focus on decreasing triatomine abundance in domestic settings. After the triatomine bugs reach a certain carrying capacity, only the speed of T. cruzi transmission will be impacted by interventions that fall short of severely reducing the population and preventing its subsequent re-infestation.
Our results suggest that the two strategies (IRS and housing improvements) for meeting the 2020 goal of interrupting domestic vector-borne transmission, could theoretically achieve this goal. This would require a centralized and sustained campaign to employ these methods in a large enough number of triatomine-infested dwellings across all 21 countries with vector-borne T. cruzi transmission, which may be extremely difficult to achieve, as there are a number of challenges in performing such an operation. These challenges include the lack of a centralized agency with the willingness and the resources to organize such an extensive campaign against Chagas disease. This in turn is further complicated by the decentralization of vector-borne disease control programs in many countries [46, 47]; the existence of many Chagas-endemic areas located in armed conflict zones (O. Cantillo and M. Vera, pers. communication [Colombia]); and competition for funding with other better-known vector-borne diseases, such as dengue fever and Chikunguyna [46, 48]. Moreover, Chagas disease patterns are highly heterogeneous, even within the same country. With a lack of sufficient baseline prevalence and/or little to no data for many areas, designing a vector control campaign with a far enough reach to eliminate Chagas disease transmission by 2020 (i.e., within the next three years) seems extremely difficult. Given these obstacles, other prevention and control measures should continue to be considered and developed for Chagas disease (e.g., early diagnosis, new medications and vaccines, etc.). Studies have shown that a Chagas vaccine could be cost-effective and could even garner a positive return-on-investment fairly early after its introduction [49, 50].
Future developments
All models are simplifications of real-life and therefore cannot account for every possible event or outcome [51]. Our results are intended to be broad, and do not account for the enormous amount of variability found in every aspect of T. cruzi transmission, including variation in parasite strain, the health status of each individual host upon infection, variation in triatomine species efficiency as T. cruzi vectors, and variation in the level and duration of T cruzi parasitemias found across different mammal species. Additionally,diversity in T. cruzi competence between animal species no doubt adds complexity to Chagas disease dynamics. For instance, individual animals that sustain a high parasitemia for relatively long periods of time can amplify T. cruzi, as has been observed in Peruvian guinea pigs [14]. Moreover, the incorporation of triatomine host preference will shift the dynamics of the system and in some scenarios could lower the human-triatomine contact rate, which has been predicted to occur under some circumstances in the presence of dogs and chickens [11]. There will also be environmental variability due to geographic location, in addition to cultural diversity that will influence human behavior. All of these are important factors to keep in mind for future models of T. cruzi transmission.
Conclusion
Our results suggest that in domestic T. cruzi transmission scenarios where no vector control measures are in place, a reduction in synanthropic animals may slow T. cruzi transmission to humans, but it would not lead to the complete interruption of transmission. We found that it is more critical to target vector abundance than synanthropic animals, and, in scenarios where measures are taken to control triatomine population growth, synanthropic animals could play a beneficial role by decreasing the speed of T. cruzi transmission to humans, and increasing the sensitivity of the system to vector control measures. More work is needed to quantify the extent of this effect in different transmission scenarios, and we do not recommend adding synanthropic animals to any system before this is studied further. Therefore, to reach the 2020 goal of interrupting intra-domiciliary T. cruzi transmission, control measures must continue to aggressively target domestic vector populations.
Abbreviations
- T. cruzi :
-
Trypanosoma cruzi
- WHO:
-
World Health Organization
- R. prolixus :
-
Rhodnius prolixus
- IRS:
-
Indoor residual spraying
References
World Health Organization. Chagas disease in Latin America: an epidemiological update based on the 2010 estimates. Wkly Epidemiol Rec. 2015;6:33–44.
WHO. Working to Overcome the Global Impact of Neglected Tropical Diseases First WHO Report on Neglected Tropical Diseases. 2010.
Lee BY, Bacon KM, Bottazzi ME, Hotez PJ. Global economic burden of Chagas disease: a computational simulation model. Lancet Infect Dis. 2013;13:342–8.
WHA63.20. WHO Eighth Plentary Meeting, 21 May 2010-Committee A, Fifth Report. 2010.
Targets and Milestones for Overcoming Neglected Tropical Diseases 2011–2020 [http://www.who.int/chagas/strategy/milestones/en/]
Dumonteil E, Ruiz-Piña H, Rodriguez-Félix E, Barrera-Pérez M, Ramirez-Sierra MJ, Rabinovich JE, et al. Re-infestation of houses by Triatoma dimidiata after intradomicile insecticide application in the Yucatán Peninsula, Mexico. Mem Inst Oswaldo Cruz. 2004;99:253–6.
Aché A, Matos AJ. Interrupting Chagas disease transmission in Venezuela. Rev Inst Med Trop Sao Paulo. 2001;43:37–43.
Espinoza N, Borrás R, Abad-Franch F. Chagas Disease Vector Control in a Hyperendemic Setting: The First 11 Years of Intervention in Cochabamba, Bolivia. PLoS Negl Trop Dis. 2014;8.
Mott KE, Mota EA, Sherlock Í, Hoff R, Muniz TM, Oliveira TS, et al. Trypanosoma cruzi infection in dogs and cats and household seroreactivity to T. cruzi in a rural community in northeast Brazil. Am J Trop Med Hyg. 1978;27:1123–7.
Cohen JE, Gürtler RE. Modeling household transmission of American trypanosomiasis. Science. 2001;293:694–8.
Gürtler RE, Cecere MC, Vázquez-Prokopec GM, Ceballos L, Gurevitz JM, Fernández MDP, et al. Domestic Animal Hosts Strongly Influence Human-Feeding Rates of the Chagas Disease Vector Triatoma infestans in Argentina. PLoS Negl Trop Dis. 2014;8:e2894.
Bezerra CM, De Góes Cavalcanti LP, De Cássia Moreira De Souza R, Barbosa SE, Das Chagas Xavier SC, Jansen AM, et al. Domestic, peridomestic and wild hosts in the transmission of Trypanosoma cruzi in the Caatinga area colonised by Triatoma brasiliensis. Mem Inst Oswaldo Cruz. 2014;109Bez:887–98.
Ramsey JM, Gutiérrez-Cabrera AE, Salgado-Ramírez L, Peterson AT, Sánchez-Cordero V, Ibarra-Cerdeña CN. Ecological Connectivity of Trypanosoma cruzi Reservoirs and Triatoma pallidipennis Hosts in an Anthropogenic Landscape with Endemic Chagas Disease. PLoS One. 2012;7(9):e46013.
Levy MZ, Tustin A, Castillo-Neyra R, Mabud TS, Levy K, Barbu CM, et al. Bottlenecks in domestic animal populations can facilitate the emergence of Trypanosoma cruzi, the aetiological agent of Chagas disease. Proc R Soc B Biol Sci. 2015;282.
Bustamante DM, De Urioste-Stone SM, Juarez JG, Pennington PM. Ecological, social and biological risk factors for continued Trypanosoma cruzi transmission by Triatoma dimidiata in Guatemala. PLoS One. 2014;9.
Lauricella MA, Sinagra AJ, Paulone I, Riarte AR, Segura EL. Natural Trypanosoma cruzi infection in dogs of endemic areas of the Argentine Republic. Rev Inst Med Trop Sao Paulo. 1989;31:63–70.
Gurtler RE, Cardinal MV. Reservoir host competence and the role of domestic and commensal hosts in the transmission of Trypanosoma cruzi. Acta Trop. 2015;52:461–8.
R. Core. Team: R. A Language and Environment for Statistical Computing. Vienna, Austria: R Foundation for Statistical Computing; 2014.
Soetaert K, Petzoldt T, Woodrow Setzer R. Solving Differential Equations in R: Package deSolve. J Stat Softw. 2010;33:1–25.
Peterson JK, Graham AL, Dobson AP, Triana O. Rhodnius prolixus fitness when when co-infected with Trypanosoma cruzi and T. rangeli. 2015. In prep.
Peterson JK, Graham AL, Dobson AP, Chavez OT. Rhodnius prolixus Life History Outcomes Differ when Infected with Different Trypanosoma cruzi I Strains. Am J Trop Med Hyg. 2015;93:564–72.
Elliot SL, Rodrigues JDO, Lorenzo MG, Martins-Filho O, Guarneri A. Trypanosoma cruzi, Etiological Agent of Chagas Disease, Is Virulent to Its Triatomine Vector Rhodnius prolixus in a Temperature-Dependent Manner. PLoS Negl Trop Dis. 2015;9, e0003646.
Stearns S. Life Histoy Tactics: A Review of the Ideas. Q Rev Biol. 1976;51:3–47.
Rassi Jr A, Rassi A, Marin-Neto JA. Chagas disease. Lancet. 2010;375:1388–402.
Das Neves Pinto AY, Aldo Valente S, Da Costa Valente V, Gomes Ferreira A, Coura JR. Fase aguda da doença de Chagas na Amazônia brasileira. Estudo de 233 casos do Pará, Amapá e Maranhão observados entre 1988 e 2005. Rev Soc Bras Med Trop. 2008;41:602–14.
Bern C. Chagas’ Disease. N Engl J Med. 2015;373:456–66.
Gurtler RE, Cecere MC, Castañera MB, Canale D, Lauricella MA, Chuit R, et al. Probability of infection with Trypanosoma cruzi of the vector Triatoma infestans fed on human and dogs in northwest Argentina. Am J Trop Med Hyg. 1996;55:24–31.
Diekmann O, Heesterbeek J, Metz J. On the definition and the computation of the basic reproduction ratio R0 in models for infectious diseases in heterogeneous populations. J Math Biol. 1990;28:365–82.
Guhl F, Aguilera G, Pinto N, Vergara D. Actualización de la distribución geográfica y ecoepidemiología de la fauna de triatominos (Reduviidae: Triatominae) en Colombia. Biomedica. 2007;27:143–62.
Enriquez GF, Bua J, Orozco MM, Wirth S, Schijman G, Gürtler RE, et al. High levels of Trypanosoma cruzi DNA determined by qPCR and infectiousness to Triatoma infestans support dogs and cats are major sources of parasites for domestic transmission. Infect Genet Evol. 2014;25:36–43.
Bowman NM, Kawai V, Levy MZ, Cornejo Del Carpio JG, Cabrera L, Delgado F, et al. Chagas disease transmission in periurban communities of Arequipa, Peru. Clin Infect Dis. 2008;46:1822–8.
Waleckx E, Gourbière S, Dumonteil E. Intrusive versus domiciliated triatomines and the challenge of adapting vector control practices against Chagas disease. Mem Inst Oswaldo Cruz. 2015;110:00.
Rabinovich JE, Himschoot P. A population-dynamics simulation model of the main vectors of Chagas’ Disease transmission, Rhodnius prolixus and Triatoma infestans. Ecol Modell. 1990;52:249–66.
Spagnuolo AM, Shillor M, Kingsland L, Thatcher A, Toeniskoetter M, Wood B. A logistic delay differential equation model for Chagas disease with interrupted spraying schedules. J Biol Dyn. 2012;6:377–94.
Monroy C, Rodas A, Mejía M, Rosales R, Tabaru Y. Epidemiology of Chagas disease in Guatemala: infection rate of Triatoma dimidiata, Triatoma nitida and Rhodnius prolixus (Hemiptera, Reduviidae) with Trypanosoma cruzi and Trypanosoma rangeli (Kinetoplastida, Trypanosomatidae). Mem Inst Oswaldo Cruz. 2003;98:305–10.
Cecere MC, Canale DM, Gürtler RE. Effects of refuges on the population dynamics of Triatoma infestans in experimental huts under natural climatic conditions in central Argentina. J Appl Ecol. 2003;40:742–56.
Dobson A. Population dynamics of pathogens with multiple host species. Am Nat. 2004;164(Suppl):S64–78.
Keesing F, Holt RD, Ostfeld RS. Effects of species diversity on disease risk. Ecol Lett. 2006;9:485–98.
Wood CL, Lafferty KD, DeLeo G, Young HS, Hudson PJ, Kuris AM. Does biodiversity protect humans against infectious disease? Ecology. 2014;95:817–32.
Gottdenker NL, Chaves LF, Calzada JE, Saldaña A, Carroll CR. Host Life History Strategy, Species Diversity, and Habitat Influence Trypanosoma cruzi Vector Infection in Changing Landscapes. PLoS Negl Trop Dis. 2012;6, e1884.
Friend WG, Choy C, Cartwright E. The Effect of Nutrient Intake on the Development and the Egg Production of Rhodnius prolixus Stahl (Hemiptera: Reduviidae). Can J Zool. 1965;43:891–904.
Gürtler RE, Kitron U, Cecere MC, Segura EL, Cohen JE. Sustainable vector control and management of Chagas disease in the Gran Chaco, Argentina. Proc Natl Acad Sci U S A. 2007;104:16194–9.
Rabinovich JE, Leal JA, Feliciangeli de Piñero D. Domiciliary biting frequency and blood ingestion of the Chagas’s disease vector Rhodnius prolixus Stahl (Hemiptera: Reduviidae), in Venezuela. Trans R Soc Trop Med Hyg. 1979;73:272–83.
Friend WG, Smith JJ. Factors affecting feeding by bloodsucking insects. Annu Rev Entomol. 1977;22:309–31.
Schofield CJ. The behaviour of Triatominae (Hemiptera: Reduviidae). Bull Entomol Res. 1979;69:363–79.
Abad-Franch F, Diotaiuti L, Gurgel-Gonçalves R, Gurtler RE. On bugs and bias: improving Chagas disease control assessment. Mem Inst Oswaldo Cruz. 2014;109:125–30.
UNICEF/UNDP/World Bank/WHO SP for R& T in TD (TDR). Descentralización Y Gestión Del Control de Enfermedades Transmisibles En América Latina. Buenos Aires: Organiza-ción Panamericana de la Salud; 2006.
Abad-Franch F, Diotaiuti L, Gurgel-Gonçalves R, Gürtler RE. Certifying the interruption of Chagas disease transmission by native vectors: cui bono? Mem Inst Oswaldo Cruz. 2013;108:251–4.
Lee BY, Bacon KM, Connor DL, Willig AM, Bailey RR. The potential economic value of a Trypanosoma cruzi (Chagas disease) vaccine in Latin America. PLoS Negl Trop Dis. 2010;4:1–8.
Lee BY, Bacon KM, Wateska AR, Bottazzi ME, Dumonteil E, Hotez PJ. Modeling the economic value of a Chagas disease therapeutic vaccine. Hum Vaccines Immunother. 2012;8:1293–301.
Lee BY. Digital decision making: computer models and antibiotic prescribing in the twenty-first century. Clin Infect Dis. 2008;46:1139–41.
Arévalo A, Carranza JC, Guhl F, Clavijo JA, Vallejo GA. Comparación del ciclo de vida de Rhodnius colombiensis Moreno, Jurberg & Galvão, 1999 y Rhodnius prolixus Stal, 1872 (Hemiptera, Reduviidae, Triatominae) en condiciones de laboratorio. Biomedica. 2007;e27(1):119–29.
Kribs-Zaleta C. Estimating contact process saturation in sylvatic transmission of Trypanosoma cruzi in the United States. PLoS Negl Trop Dis. 2010;4.
Organization PAH. Health in the Americas: 2012 Edition. Washington DC: Regional Outlook and Country Profiles; 2012.
Schenone H, Rojo M, Cocha L. Positividad diurna y nocturna del xenodiagnostico en un paciente con infeccion chagasia cronica de parasitemia permanente. Bol Chil Parasitol. 1977;33:63–6.
Perlowagora-Szumlewicz A, Muller CA. Studies in search of a suitable experimental insect model for xenodiagonsis of hosts with Chagas’ disease. 2-Attempts to upgrade the reliability and the efficacy of xenodiagnosis in chronic Chagas’ disease. Mem Inst Oswaldo Cruz. 1987;82:259–72.
Nouvellet P, Dumonteil E, Gourbière S. The Improbable Transmission of Trypanosoma cruzi to Human: The Missing Link in the Dynamics and Control of Chagas Disease. PLoS Negl Trop Dis. 2013;7, e2505.
Rabinovich J, Schweigmann N, Yohai V, Wisnivesky-Colli C. Probability of Trypanosoma cruzi transmission by Triatoma infestans (Hemiptera: Reduviidae) to the opossum Didelphis albiventris (Marsupialia: Didelphidae). Am J Trop Med Hyg. 2001;65:125–30.
Catala SS, Gorla DE, Basombrio MA. Vectorial transmission of Trypanosoma cruzi: an experimental field study with susceptible and immunized hosts. Am J Trop Med Hyg. 1992;47:20–6.
Acknowledgements
The authors gratefully acknowledge funding from the NTD Modelling Consortium by the Bill and Melinda Gates Foundation in partnership with the Task Force for Global Health. APD and JKP also acknowledge Princeton Grand Challenges Program in Global Health and continuous lively discussions with the Infectious Disease Group at EEB, Princeton University. The views, opinions, assumptions or any other information set out in this article are solely those of the authors.
Author information
Authors and Affiliations
Corresponding author
Additional information
Competing interests
The authors declare that they have no competing interests.
Authors’ contributions
JKP contributed to the model conception, design, and development; designed model experiments; collected input data; performed model runs; analyzed and interpreted the results; and drafted the manuscript. SMB helped design model experiments; collected input data; analyzed and interpreted the results; and helped draft the manuscript. BYL interpreted the results and critically revised the manuscript. APD contributed to the model conception, design, and development; helped design model experiments; analyzed the results; and critically revised the manuscript. All authors have read and approved the submitted manuscript.
Rights and permissions
Open Access This article is distributed under the terms of the Creative Commons Attribution 4.0 International License (http://creativecommons.org/licenses/by/4.0/), which permits unrestricted use, distribution, and reproduction in any medium, provided you give appropriate credit to the original author(s) and the source, provide a link to the Creative Commons license, and indicate if changes were made. The Creative Commons Public Domain Dedication waiver (http://creativecommons.org/publicdomain/zero/1.0/) applies to the data made available in this article, unless otherwise stated.
About this article
Cite this article
Peterson, J.K., Bartsch, S.M., Lee, B.Y. et al. Broad patterns in domestic vector-borne Trypanosoma cruzi transmission dynamics: synanthropic animals and vector control. Parasites Vectors 8, 537 (2015). https://doi.org/10.1186/s13071-015-1146-1
Received:
Accepted:
Published:
DOI: https://doi.org/10.1186/s13071-015-1146-1