Abstract
Neuroinflammation is an important hallmark of amyotrophic lateral sclerosis (ALS) and frontotemporal lobar degeneration (FTLD). An inflammatory reaction to neuronal injury is deemed vital for neuronal health and homeostasis. However, a continued activation of the inflammatory response can be detrimental to remaining neurons and aggravate the disease process. Apart from a disease modifying role, some evidence suggests that neuroinflammation may also contribute to the upstream cause of the disease. In this review, we will first focus on the role of neuroinflammation in the pathogenesis of chromosome 9 open reading frame 72 gene (C9orf72) hexanucleotide repeat expansions (HRE)-mediated ALS/FTD (C9-ALS/FTD). Additionally, we will discuss evidence from ex vivo and in vivo studies and finally, we briefly summarize the trials and progress of anti-inflammatory therapies.
Similar content being viewed by others
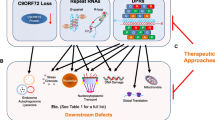
Background
Amyotrophic lateral sclerosis (ALS) and frontotemporal lobar degeneration (FTLD) are adult-onset progressive neurodegenerative diseases that are part of the same disease spectrum [1]. Both diseases are characterized by the accumulation of neurotoxic protein depositions, consisting of TAR DNA binding protein (TDP-43) in > 95% of ALS cases and about 50% of FTLD cases [2, 3]. The neuroanatomical distribution of neuropathological lesions varies and correlates with the clinical presentation. In ALS, the motor system is primarily affected with degeneration of motor neurons (MNs) in the motor cortex, brainstem and spinal cord, while in FTLD, the frontal and anterior temporal cortex are mostly affected. The clinical phenotype of ALS is characterized by a combination of upper and lower motor involvement leading to muscle weakness, hyperreflexia, spasticity, as well as muscle atrophy and fasciculations. The disease typically has a focal onset, most commonly with unilateral distal weakness in one limb or tongue weakness, but has a tendency to spread to other body regions [4,5,6,7]. FTLD patientspresent with a clinical syndrome of frontotemporal dementia (FTD), a subtype of dementia recognizable primarily by either changes in behaviour (behavioral variant FTD (bvFTD)) and deficits in language (primary progressive aphasia (PPA)), or both [8,9,10]. In approximately 10% of ALS cases and 40% of FTD cases, the family history is positive and such patients are classified as familial ALS (fALS) or familial FTD (fFTD), respectively. It is mainly transmitted via an autosomal dominant Mendelian inheritance pattern. The remaining cases are classified as sporadic disease (sALS or sFTD) [7]. Significant overlap exists between ALS and FTD, as 10–15% of ALS patients also develop FTD and a similar proportion of FTD patients also develop motor problems during the course of their disease [1, 4, 11,12,13]. A common genetic basis also exists. The most common cause of both fALS (40%) and fFTD (25%) [14, 15] are GGGGCC hexanucleotide repeat expansions (HRE) in the 5’ non-coding region of chromosome 9 open reading frame 72 gene (C9orf72) [14,15,16], which will be the focus of this review. How C9orf72 HRE cause neurodegeneration is only partially understood and several disease mechanisms have been put forward. The neurodegenerative process is accompanied by neuroinflammation, similar to what is observed in other neurodegenerative diseases [17]. Such an inflammatory reaction to neuronal damage is deemed to be important and may contribute to the disease pathogenesis. In this review, our aim is to characterize the role of neuroinflammation in the pathogenesis of C9-ALS/FTD and relate this to the different disease mechanisms proposed for these diseases.
C9orf72-mediated ALS/FTD
Mutations in the C9orf72 gene are inherited as an autosomal dominant disease with incomplete penetrance [18, 19]. Some C9orf72 HREs carriers (C9-carriers) develop the disease at an early age while in other carriers the disease doesn’t manifest before their ninth decade of life. By the age of 58, about 50% of carriers will have ALS or FTD [18].
The exact number of repeats sufficient to cause C9-ALS/FTD has not been strictly determined, and consequently, more studies are needed. Currently, a repeat number of 30 is regularly used as threshold. This makes sense, since most patients have hundreds to thousands of repeats. Nonetheless, some reports of ALS and FTD cases with only 20–30 repeats exist [19,20,21,22,23,24,25] and it has also been suggested that carrying an intermediate expansion on both alleles might contribute to ALS [26].
Interestingly, repeat sizes can vary between family members with C9-ALS/FTD, even between monozygotic twins [24, 27]. On top of that, the instability of the expansion also causes a variation in repeat sizes of the expansion between different tissues within the same individual. This way, the repeat size measured in blood may differ from the repeat size present in brain [23, 28,29,30]. A correlation between repeat size and age of onset, disease progression rate and survival has been suggested, but remains unclear [24, 31,32,33]. The association between repeat size and age of onset is potentially biased by the confounding factor of age at sample collection [24].
Pathological characterization of C9-ALS/FTD
Post-mortem brain and spinal cord tissues of C9-ALS/FTD patients revealed some highly characteristic pathological features in addition to the TDP-43 pathological lesions, including RNA foci and protein aggregates containing dipeptide repeats (DPRs) and the autophagy adaptor protein p62/sequestosome1 (p62) [34, 35].
The presence of RNA foci, consisting of sense and anti-sense G4C2 repeat RNA, the spliced intron 1 of the gene, or the complete C9orf72 (pre-)messenger RNA (mRNA), has been described in C9-ALS/FTD brain and spinal cord tissues [14, 27, 36]. These RNA foci are predominantly localized in neuronal nuclei in the frontal and motor cortices, hippocampus, cerebellum and in the spinal cord [37]. They have also been found in induced pluripotent stem cell (iPSC)-derived motor and cortical neurons, fibroblasts and sometimes also in patient-derived astrocytes, microglia and oligodendrocytes [38,39,40].
Another pathologic hallmark of C9-ALS/FTD is the presence of toxic DPRs. These are produced by repeat-associated non-AUG (RAN) translation of C9orf72 repeat RNA. Poly-glycine-alanine (GA) and poly-glycine-arginine (GR) DPR species are translated from sense G4C2 transcripts, while poly-proline-alanine (PA) and poly-proline-arginine (PR) proteins originate from antisense G4C2 transcripts, and poly-glycine-proline (GP) proteins may originate from both sense and antisense transcripts [36, 38, 41,42,43]. In C9-ALS/FTD, DPR inclusions are present in the cytoplasm and nucleus of neurons, mostly in hippocampus, thalamus, cortex, amygdala and the cerebellar granular layer [21, 42, 44]. These DPR-inclusions mostly stain positive for ubiquitin and p62 while they do not colocalize with TDP-43 inclusions [21].
C9-ALS/FTD is associated with TDP-43 inclusions, as is common in non-C9-ALS/FTD as well. TDP-43 pathology can be found in about 97% of ALS patients and in about 50% of FTD. [45, 46]. Recently, a study showed that poly-GR may directly sequester TDP-43 and induce TDP-43 aggregation [47]. The cytoplasmic and/or intranuclear pTDP-43 inclusions in C9-carriers are accompanied by nuclear depletion of TDP-43 and can be found in the cerebral cortex and subcortical regions such as the striatum, hippocampus, basal ganglia, and substantia nigra [48,49,50,51,52]. Some studies also reported cytoplasmic p62 and pTDP-43-positive inclusions in glial cells, such oligodendrocytes and astrocytes [35, 53].
Pathomechanism of C9-ALS/FTD
Before delving deeper into the role of neuroinflammation, we will first briefly outline the most important disease mechanisms at play in C9-ALS/FTD. Currently, there are three non-mutually exclusive hypotheses explaining the role of C9orf72 HREs in disease: (1) a loss-of-function mechanism with reduced levels of C9orf72 transcripts causing a reduction of C9orf72 protein levels, better known as C9orf72 haploinsufficiency; (2) a toxic gain-of-function mechanism with the formation of RNA foci, in which sense and antisense HRE RNAs sequester RNA-binding proteins and other proteins; (3) a toxic gain-of-function mechanism consisting of RAN translation of the sense and antisense HRE RNA resulting in toxic DPRs. These disease mechanisms are summarized in Fig. 1.
Overview of the different disease mechanisms at play in C9orf72-ALS/FTD. First, the (GGGGCC)n hexanucleotide repeat expansion could reduce transcription of the C9orf72 gene leading to reduced C9orf72 protein levels (haploinsufficiency) that may ultimately cause dysfunction of the autophagy-lysosome pathway. Second, bidirectional transcription of the repeat expansion forms sense (GGGGCC) and antisense (CCCCGG) RNA transcripts that form secondary structures and may cause RNA toxicity by sequestering essential RNA-binding proteins (RBPs). Finally, unconventional repeat-associated non-ATG (RAN) translation produces toxic dipeptide repeat proteins (DPRs): polyGP, polyGR and polyGA from the sense strand and polyGP, polyPR and polyPA from the antisense strand. These toxic DPRs are able to form aggregates and may affect several essential cellular pathways such as mitochondrial function, axonal transport, proteasome function and protein translation. Moreover, these three mechanisms may work separately or in synergy to cause alterations in nuclear-cytoplasmic transport, TDP-43 subcellular localization and stress granule dynamics that are toxic to motor neurons. DPR: dipeptide repeat protein; RAN: repeat associated non-ATG translation; RBP: RNA-binding protein; TDP-43: TAR DNA-binding protein 43
Loss of function
The C9orf72 gene is located on chromosome 9p21 and consists of 11 exons. While pre-mRNA transcript variants 2 and 3 encode a C9orf72-long protein (isoform A, 481 amino acids, exons 2–11), the transcript variant 1 encodes a C9orf72-short protein (isoform B, 222 amino acids, exons 2–5) [54]. In human iPSC-derived MNs the long isoform is localized to lysosomes and pre-synapses while the short isoform is localized along the nuclear membrane [55, 56]. These findings imply that they might have different cellular functions. It has been suggested that the long isoform is involved in the autophagy-lysosome pathway, while short isoform may play a role in nucleoplasmic transport [57, 58]. In fact, multiple studies conducted in a variety of (neuronal) cell lines, iPSC-derived neurons and rodent models of ALS found a link between C9orf72 haploinsufficiency and alterations in the endo-lysosomal pathway [57].
Although a reduction of some of the C9orf72 transcripts has been found in blood lymphocytes, iPSC-derived neurons, cerebellum, frontal cortex, motor cortex and spinal cord of C9-carriers [59], several other studies could not confirm this and, as a result, the C9orf72 haploinsufficiency hypothesis still remains debated in the field [60, 61]. Reduced C9orf72 protein levels have been reported in the cerebellum of C9-carriers, but to what extent C9orf72 protein levels are reduced in C9-ALS/FTD remains unclear [55]. Some of the C9orf72 antibodies used have been criticized for their lack of specificity [62]. Although reduced levels of some of the C9orf72 transcripts and reduced protein levels have been described in some studies, this could not been confirmed in other studies. The contribution of reduced C9orf72 function in ALS/FTD requires further study. As such, the role of C9orf72 haploinsufficiency in C9-ALS/FTD remains debated. Several reviews on this topic have been recently published [57, 58, 63].
RNA gain of function
The mechanism of RNA gain-of-function is well-established in other repeat diseases [40, 64]. The G4C2 HRE in the C9orf72 gene can form multiple secondary structures which can bind and sequester several proteins. Both sense and antisense transcripts, containing GGGGCC or CCCCGG repeats, respectively, are being formed and identified in RNA foci [14, 65]. In most C9orf72 model systems, sense repeat RNA foci are easier to detect and more abundant than their antisense counterparts. Nevertheless, antisense foci can be robustly detected in several cell types and post-mortem samples [66].
The sense strand of the G4C2 repeat is capable of forming G-quadruplexes both at the DNA and RNA level [67,68,69]. G-quadruplexes are abundant in promoter and intronic regions of several genes and they are involved in multiple biological processes such as transcription, alternative splicing, translation regulation, genetic instability, telomere regulation and RNA transport and degradation [69,70,71]. Transcribed G4C2 RNA can bind to G4C2 repeat DNA and form R-loops, a three-stranded structure formed by a DNA/RNA hybrid, resulting in disruption of transcription and genome instability [68, 72].
Repeat-containing RNA can sequester several RNA-binding proteins and influence their function. Binding of important cellular proteins to C9orf72 repeat RNA or their sequestration in RNA foci results in depletion of these proteins which dysfunction of multiple cellular processes. The largest group of RNA-binding protein known to bind the G4C2 repeat RNA is the heterogeneous nuclear ribonucleoprotein group (hnRNP). Sequestration of hnRNPs causes splicing alterations of several genes [40]. The role of repeat RNA toxicity is reviewed in detail elsewhere [64].
DPR toxicity
The impact of DPRs on cellular functioning has been studied extensively. We will only briefly touch upon the different pathways that are altered by DPR expression as excellent reviews focussing on RAN translation and DPR toxicity exist [73, 74].
In general, the toxicity of the different DPRs varies, the arginine-containing DPRs, poly-GR and poly-PR, are found to be more toxic than the non arginine-containing DPRs, poly-GA, poly-PA and poly-GP [59]. The arginine-containing DPRs are capable of binding proteins that harbor low-complexity domains (LCDs) [75, 76]. As such, these arginine-containing DPRs may alter liquid–liquid phase separation, a process crucial for the formation of stress granules (SGs) [77,78,79]. On top of that, poly-GR and poly-PR can also affect nucleocytoplasmic transport by altering the splicing of RNA protein Ran-Gap, by binding of importins, nucleoporins (Nup) or the lamin B receptor, or by disturbing SG dynamics [80,81,82]. DPRs also cause nucleolar stress, leading to splicing and mRNA translation defects. Studies have demonstrated that poly-GR and poly-PR can bind several RNA-binding proteins, nuclear proteins, hnRNPs, ribosomal proteins and spliceosome components and subsequently disrupt global protein translation and RNA metabolism [78, 79, 83]. Furthermore, arginine-containing DPRs were recently found to interfere with the axonal transport machinery by binding to tubulin and motor proteins in iPSC-derived motor neurons [61, 84]. Poly-GR, as well as other DPRs, are also linked to mitochondrial and DNA damage. Feature of DNA damage and oxidative stress have been found in iPSC-derived MNs of C9-ALS/FTD patients [85]. Lastly, poly-GA, the most abundant DPR, probably acts by blocking the ubiquitin–proteasome system (UPS) as expression of poly-GA in HEK293T, neuro2a cells and primary mouse cortical neurons led to an elevated p62 expression and accumulation of ubiquitinated proteins [86].
Major inflammatory pathways
Before reviewing the role of neuroinflammation in C9-ALS/FTD, we will shortly discuss the intracellular signaling pathways that are activated in glial cells in neuroinflammation. These three pathways are illustrated in Fig. 2.
Major inflammatory pathways. A The JAK-STAT pathway regulates the cellular response to cytokines and growth factors and signals through dimerization of receptors upon binding of these molecules. Upon dimerization, a series of autophosphorylation and transphosphorylation events of the receptors and their associated JAK proteins results in an active STAT dimer that functions as transcription activator. B The MAPK and NF-κB pathway is characterized by a multi-step signaling cascade upon receptor activation eventually resulting in MAPK activation. In this cascade, generally a MAPK kinase kinase (MAP3K) phosphorylates and activates a MAPK kinase (MAP2K) which in turn activates the MAPK. Meanwhile, the MAP3K can also induce NF-κB signaling. Together with the transcription factor activator protein 1 (AP-1) activated by phosphorylation of the MAPK, NF-κB translocates to the nucleus and functions as a transcription factor. C The NLRP3 inflammasome pathway can be initiated by a plethora of different mechanisms that result in activation of the NF-κB pathway. The presence of additional cellular insults will result in the formation of the NLRP3 inflammasome which is able to activate caspase 1. The activated caspase 1 dimer is subsequently able to process gasdermin D, pro-IL-1β and pro-IL-18 into their mature forms causing the formation of a gasdermin D pore resulting in pyroptosis and the release of inflammatory cytokines. AP-1: activator protein 1; ATP: adenosine triphosphate; ASC: apoptosis associated speck-like protein containing a CARD; DAMP: damage-associated molecular pattern; IκBα: nuclear factor of kappa light polypeptide gene enhancer in B-cells inhibitor α; IKKα/β: Inhibitor of nuclear factor kappa-B kinase subunit alpha α/β; IL: interleukin; IL1-R: interleukin-1 receptor; IRAK1/4: interleukin-1 receptor-associated kinase 1/4; JAK: janus kinase; JNK: c-Jun N-terminal kinase; MAP2Ks: mitogen-activated protein kinase kinases; mtDNA: mitochondrial DNA; mtROS: mitochondrial reactive oxygen species; MyD88: myeloid differentiation primary response 88; NEK7: NIMA-related kinase 7; NEMO: NF-κB essential modulator; NF-κB: nuclear factor kappa-light-chain-enhancer of activated B cells; NLRP3: NLR family pyrin domain containing 3; P2X4/7: ATP-gated P2X receptor cation channel 4/7; p38: p38 mitogen-activated protein kinase; PAMP: pathogen-associated molecular pattern; STAT: signal transducer and activator of transcription; TAB1,2,3: TGF-β activated kinase 1 binding protein 1, 2 or 3; TAK1: TGF-β activated kinase 1; TLR: toll-like receptor; TRAF6: TNF receptor associated factor 6
The Janus kinase (JAK)-signal transducer and activator of transcription (STAT)
The JAK/STAT pathway plays a critical role in the regulation of the inflammatory response. This pathway is activated by cellular responses to cytokines (including interferons and interleukins) and growth factors [87,88,89]. Binding of these molecules to their cell surface receptors leads to dimerization of the receptor and hence brings the receptor-associated JAK proteins in close proximity. This enables the JAKs to transphosphorylate each other, enhancing their activity. The JAKs also phosphorylate tyrosines on the intracellular domains of the cytokine receptor itself, generating binding sites for inactive STAT proteins. Upon binding, the STAT proteins themselves also get phosphorylated by the JAKs which leads to their dissociation from the receptor and subsequent dimerization/oligomerization. In this state, the STATs are translocated to the nucleus, and regulate the transcription of their target genes which comprise mostly pro-inflammatory factors. Among STATs, STAT3 may be very important in microglia and astrocytes with effects on other cell types [90,91,92,93,94,95].
Mitogen-activated protein kinase (MAPK) and nuclear factor kappa light chain enhancer of activated B cells (NF-κB)
MAPKs are serine–threonine kinases that are involved in signal transduction of multiple extracellular stimuli including growth factors, pro-inflammatory cytokines and mitogens [96]. The MAPK pathway has been shown to be involved in several neurological conditions [97,98,99]. The typical and major MAPKs are characterized by multiple downstream phosphorylation events upon receptor activation ultimately leading to the activation of the MAPK. In mammals, the p38 kinases, c-JUN N-terminal kinases (JNKs) and extracellular signal-regulated kinases (ERKs) are the three major MAPK families. While the ERK family of MAPKs is responsible for differentiation and proliferation signalling upon growth factor and mitogen binding, the JNKs and p38 kinases (highly expressed in glial and neuronal cells) are stress-activated protein kinases primarily activated through various environmental stresses and cytokines [100]. Upon binding of the signalling molecules to various receptors, a multi-step signalling cascade is initiated. In many cases, myeloid differentiation primary response protein 88 (MyD88) recruits interleukin-1 receptor-associated kinase-4 (IRAK4). This complex will subsequently recruit, phosphorylate and activate other IRAKs (e.g. IRAK1 and IRAK2). Activated IRAKs are able to activate TNF receptor-associated factor 6 (TRAF6) which in turn activates the complex composed of transforming growth factor-β-activated kinase 1 (TAK1) (a MAPK kinase kinase or MAP3K) and TGF-beta activated kinase 1 binding protein 2 and 3 (TAB2/3). This complex can then activate MAPK kinases (MAP2Ks), but also induce the NF-κB signalling pathway. On its turn, MAP2K phosphorylates the p38 or c-Jun N-terminal kinase (JNK) MAPKs, which results in the production of the transcription factor activator protein 1 (AP-1). Finally, the NF-κB and activator protein 1 (AP-1) transcription factors translocate to the nucleus and activate the transcription of cytokine inducible genes.
NOD-like receptor family pyrin domain containing 3 (NLRP3) inflammasome
The first step in NLRP3-mediated inflammation is triggered by the binding of pathogen-associated molecular pattern molecules (PAMPs) or damage-associated molecular pattern molecules (DAMPs) to Toll-like receptors (TLRs) or nucleotide-binding oligomerization domain and leucine-rich repeat-containing receptors (NLRs), or by the binding of endogenous cytokines to their respective receptors ultimately activating the transcription factor of NF-κB signalling pathway. This, in turn, results in the increased transcription of the NLRP3, pro-IL-1β and pro-IL-18, two pro-inflammatory cytokines. The second step in the NLRP3 inflammasome activation cascade requires some kind of cellular insult such as mitochondrial damage, reactive oxygen species (ROS) generation, lysosomal dysfunction or ionic flux dysregulation. Ultimately, this will result in the interaction of NLRP3 and apoptosis associated speck-like protein (ASC), inducing caspase 1 activation. This leads to cleavage, maturation and eventually the secretion of IL-1β and IL-18, mediating inflammation and the innate immune response [101, 102]. In addition, active caspase 1 also cleaves the protein gasdermin D, which enables it to oligomerize and form a gasdermin D membrane pore. This causes pyroptosis, an inflammatory mediated cell death that is triggered by proinflammatory molecules and occurs as the result of membranous pore formation, cytoplasmic swelling, and leakage of cytosolic contents [103, 104].
Neuroinflammation in C9-ALS/FTD
Even though the exact role of neuroinflammation in neurodegeneration still remains debated, dysregulation of the immune system is a pathological hallmark in nearly all neurodegenerative diseases [105,106,107]. It consists of the activation of glial cells with an increased activation of the proinflammatory MAPK and NF-κB signalling pathways, increased cell surface expression of cell adhesion molecules, increased cytosolic expression of proinflammatory enzymes and an increased release of proinflammatory secretory proteins such as cytokines, chemokines and growth factors [108,109,110,111,112]. While neuroinflammation is mainly studied in patients and in vivo models systems due to the inherent nature of the process that requires multiple cell types and secreted molecules to interact, recent advances in the field of iPSCs made it also possible to investigate some deregulated processes in relevant cell types in vitro. In addition, iPSCs also enable the study of cell autonomous mechanisms of deregulated processes as cells are naïve to interactions with other cell types. This is summarised in Fig. 3.
Overview of the neuroinflammatory pathways at play in C9-ALS/FTD. Coinciding with the neurodegeneration, astrocytes and microglia undergo a switch towards a neurotoxic and (over)activated state in which they produce and secrete an array of pro-inflammatory factors including cytokines (TNFα, IL-6, IL-12, IL-1β, TGFβ, IFNγ), glutamate, NO and ROS. During disease progression, peripheral immune cells such as T cells, B cells, monocytes and NK cells migrate into the CNS. Upon CNS infiltration, monocytes may differentiate to macrophages that act similar to the CNS-residing microglia. Although an active involvement of T cells in ALS pathophysiology is debated, disease progression has been associated with a disbalance between neuroprotective and neurotoxic T cell populations. Next, NK cells are attracted to the CNS by chemokines and can stimulate neurotoxic glia differentiation or directly be toxic to the neurons. Finally, although their role has never been directly demonstrated, activated B cells may exert toxicity by the secretion of autoimmune antibodies. CCL2: C–C motif chemokine ligand 2; CNS, central nervous system; CX3CL1: CX3 chemokine ligand 1; CXCL10: C-X-C motif ligand 10; IFNγ, interferon γ; IL, interleukin; NK cell: natural killer cell; NO, nitric oxide; ROS, reactive oxygen species; TGFβ, transforming growth factor β; TNFα, tumor necrosis factor α
Glial cells
Glial cells or neuroglia are non-neuronal cells located in the central nervous system (CNS) and are responsible for CNS homeostasis, structural and trophic support for neurons, removal of pathogens and modulating neurotransmission. While neuroglia include multiple cell types such as oligodendrocytes, satellite cells and Schwann cells, we will focus on microglia and astrocytes in this review as these cell types have been shown to have a major role in the regulation of neuroinflammation.
Microglia
Microglia are the resident innate immune cells of the CNS, making up 5–15% of all cells in the brain [113, 114]. As such, they are the first responders to pathological injuries and have a crucial role in the maintenance of homeostasis in the CNS [106, 115,116,117,118]. Microglia are able to interact with both immune cells residing in the CNS as well as with peripheral immune cells that infiltrate into the CNS resulting in multiple crosstalk events [119, 120]. Microglia exist in various forms depending on their activation status. In the early stages of neurodegenerative diseases, anti-inflammatory cytokines such as transforming growth factor beta (TGF-β), glial cell-derived neurotrophic factor (GDNF) and some interleukines (IL-4, IL-10 and IL-13) guide microglia to a neuroprotective state (M2 microglia) leading to the release of diverse neuroprotective factors such as insulin-like growth factor (IGF-1) [121, 122]. Furthermore, regulatory T-cells (Tregs) and the neuroprotective M2 microglia downregulate the proliferation and toxic function of Th1 cells and toxic microglial cells (M1 microglia). However, in a later stages of disease, glia and immune cells transform towards an opposite and more cytotoxic phenotype. This response is a result of the secretion of ROS, pro-inflammatory cytokines such as IL-1β and IL-6, tumor necrosis factor-alpha (TNF-α) and chemokines including CCL2 (C–C motif chemokine ligand 2) [121, 123]. Ultimately, this leads to the suppression of Tregs and an increased production of interferon-gamma (IFN-γ) by T helper 1 (Th1) cells [124, 125]. Taken together, upon sustained neuroinflammation, the neuroprotective microglial response switches from a protective to a neurotoxic response [116]. Eventually, the inability for neuronal repair and the dystrophic morphology of microglia will lead to synaptic impairment, oxidative damage and mitochondrial dysfunction, which in turn will result in aggravation of neurodegeneration [121, 126,127,128]. The concept of M1 and M2 microglia is an oversimplification which does not fully reflect the inflammatory cell states of microglia in neurodegenerative diseases. The recent advent of novel technologies, such as single-cell RNA sequencing, mass cytometry and proteomics, has facilitated the profiling of single cells with high-throughput technologies [129, 130]. There is increasing evidence for subclasses of microglial cell states that are seen in neurodegenerative diseases [131]. However, the heterogeneity of microglia across different CNS regions and their functionality during disease needs to be further investigated.
Cellular studies that aimed to decipher the role of C9orf72 and the consequences of its haploinsufficiency in microglia mostly pointed towards an involvement of C9orf72 in the endo-lysosomal pathway [57]. Unfortunately, the differentiation protocols for iPSC-derived microglial cells have only recently emerged and hence only limited in vitro data concerning the role of C9orf72 in microglia l cells is available [132]. One study has used C9-ALS/FTD iPSC-derived microglia and showed that microglia produce DPRs and have reduced C9orf72 protein levels [133]. Although transcriptional profiles between C9orf72 microglia and control cells were found to be highly similar with only 27 genes being differentially expressed, they did observe a dysfunction of the endo-lysosomal pathway. This was supported by changes in lysosomal associated membrane protein 1 (Lamp1) and early endosome antigen 1 (EEA1) levels, which was accompanied by defects in phagocytosis [133]. On top of that, stimulation of the inflammatory phenotype in these microglia with lipopolysaccharides (LPS) and amyloid beta (Aβ) resulted in an augmented inflammatory response [133]. Interestingly, these in vitro phenotypes closely resemble those found in microglia of postmortem tissues from C9-ALS/FTD patients [134, 135]. Another recent study explored the possible dysfunction and therapeutic potential of C9orf72 patient iPSC-derived M2 macrophages. Surprisingly, the presence of the C9orf72 HRE did not affect the immunomodulatory activity of the M2 microglial cells and still allowed them to convert blood-derived Teffs into functional Tregs and counteract the activation of M1 microglia [136].
As C9orf72 is highly expressed in myeloid cells (including microglia), the elucidation of its role in microglia l function has received a lot of attention in several knockout C9orf72 mouse models. Progressive splenomegaly and lymphadenopathy, increased levels of pro-inflammatory cytokines, autoinflammation with the production of autoantibodies, and auto-immune like diseases have all been reported in both C9orf72 + / − and C9orf72 − / − knockout mouse models [134, 137,138,139]. These findings suggest that C9orf72 haploinsufficiency is sufficient to drive an altered myeloid cell function and systemic immune response [134, 137]. Interestingly, these knockout mouse models did not display a neurodegeneration phenotype consistent with ALS/FTD, indicating that the deletion of C9orf72 alone is not sufficient to cause C9 ALS/FTD. Nevertheless, microglia-induced MN death in ALS is in part caused by downregulation of NF-kB signaling in microglia and attenuating the proinflammatory microglial activation can prolong survival in ALS mice [139,140,141]. In this last study, Zhao and colleagues found evidence for activation of the NLRP3 inflammasome pathway by TDP-43 selectively in microglia as motor neurons did not exhibit neurotoxic effects [141]. Of note, this pathway can be activated by numerous other pathological mechanisms at play in C9-ALS/FTD, such as mitochondrial damage, lysosomal dysfunction and the presence of protein aggregates. In both C9orf72 − / − macrophages and C9orf72 − / − microglia, lysosomal accumulations and increased production of circulating proinflammatory cytokines, such as IL-6 and IL-1β, as a result of hyperactive immune responses, have been reported [34]. Moreover, the spinal cord from C9orf72 -/- mice, poly-PR (GFP-PR28) heterozygous overexpression mice and Thy1 (GA)149-CFP overexpression mice all showed an upregulation of inflammatory pathways [142,143,144].
As we have discussed in detail in a recent review, the C9orf72-SMCR8-WDR41 complex is an initiator of autophagy and lysosomal function [57]. Smcr 8-/- mice have similar inflammatory phenotypes as C9orf72-/- mice consisting of splenomegaly, lymphadenopathy, activated circulating T cells and excessive inflammatory cytokine production [145]. TLRs play crucial roles in the innate immune system mediated through NF-κB and interferon regulatory factors (IRFs) signalling which result in the production of inflammatory cytokines or chemokines. In a Smcr8 −/− mice model, the autoimmunity and inflammatory phenotypes that closely resemble the ones found in C9-ALS/FTD were rescued by triple knockout of the endosomal TLRs [146].
In an adeno-associated virus (AAV) gain-of-function C9-ALS/FTD mouse model, accumulation of RNA foci and DPR proteins, TDP-43 pathology, gliosis, neurodegeneration and behavioral abnormalities have been reported [147, 148]. In poly-GA and poly-PR mouse models, the role of inflammation and TDP-43 abnormalities in neurodegeneration have been investigated [149]. By performing bulk RNA sequencing of brainstem, cortex, hippocampus and spinal cord, it was shown that poly-GA mouse models have a strong immune response including upregulation of interferon-inducible genes. At the end stage of disease, activation of an interferon pro-inflammatory microglial signature has been reported in poly-GA mice compared to poly-PR mice. These findings were also confirmed in the spinal cord of C9-ALS patients [149].
Astrocytes
Astrocytes make up another dominant cell population in the brain. Astrocytes play a significant role in the maintenance of the blood brain barrier (BBB), they provide metabolic support to neurons, aid in axonal health maintenance, protect against oxidative stress, restrict the spread of cytotoxic inflammation and regulate the neuroendothelial permeability [150,151,152,153]. Similar to microglia, astrocytes can switch from a protective to a neurotoxic activated phenotype, commonly referred to as A2 and A1 astrocytes respectively. Activated astrocytes produce and release multiple proinflammatory molecules such as cytokines, chemokines and small molecules including ROS and nitric oxide (NO), all of which are toxic to MNs. In addition, these factors can activate microglia and recruit infiltrating peripheral immune cells to the CNS further exacerbating the neurodegenerative process by suppressing neuronal supporting mechanisms [119, 154,155,156,157]. Like microglia, astrocyte heterogeneity across the CNS regions and age-dependent changes in gene expression that may contribute to neuroinflammation have been reported [158]. Nevertheless, the functional implications of these changes in gene expression and cellular motor neuron-immune cell crosstalk are still lacking.
iPSC-derived astrocyte models of C9-ALS/FTD have been around for several years now, and co-culture experiments with MNs have revealed that the toxic effects of astrocytes on motor neurons are mediated through physical contact as well as by soluble factors [159,160,161]. In one study, however, replacement of the cell culture medium by control astrocyte conditioned medium (ACM) was insufficient to reverse the MN cell death [160]. Moreover, impairment of autophagy, increased SOD1 levels and activation of inflammatory pathways, especially the NF-κB signalling pathway, might play a role in this toxicity [135, 159, 162]. Interestingly, knockdown of C9orf72 in astrocytes proved to have profound effects on astrocyte-mediated neurotoxicity as this resulted in p62 inclusion formation and major dysfunctions in glutamate homeostasis (e.g. intracellular glutamate accumulation and impaired glutamate uptake by decreased levels of the astrocytic excitatory amino acid transporter 1 and 2 (EAAT1-2)) [163]. Additional investigations led to the finding that both NFκB and the shorter C9orf72 isoform can bind to the endothelin 1 (EDN1) promotor site, a negative regulator of EAAT2 [163]. In addition, iPSC-derived astrocytes harboring SOD1 or C9orf72 mutations, and astrocytes derived from sALS patients, did exert neurotoxic effects on MNs and presented with disease-associated astrocytic phenotypes [164,165,166,167]. More specifically, these neurotoxic effects were mediated by a decrease in the magnitude of voltage‐activated Na+ and K+ currents, a decrease in the secretion of antioxidants and an increase in astrocytic oxidative stress and premature cell senescence [165]. Finally, in vivo transplantation of human astrocyte progenitor cells in the mouse spinal cord revealed that astrocytes from sporadic ALS patients induced a neuroinflammatory phenotype in these mice accompanied by ubiquitin aggregates, movement decline and a reduction in MN numbers [168].
Although several studies reported on the toxicit of C9-ALS/FTD astrocytes, comparison between these studies remains challenging due to differences in the methods used, such as the astrocyte differentiation protocols. For instance, one study generated iPSCs from fibroblasts before differentiating them into astrocytes in chemically defined media (31,841,614), while another study first generated neural precursors from adult skin fibroblasts before differentiating them into astrocytes using serum-containing media (24,379,375). As a consequence of these methodological differences, different transcriptional patterns with changes in functionality may be present in those astrocytes ( 27,725,795). Differences in the purity of cultures andthe use of primary cultures of mutant C9orf72 expressing mouse astrocytes may explain differences in study results (31,841,614). Future studies are needed to investigate the effect of mutant C9orf72 astrocytes on MNs survival. In addition, the exact role of astrocyte-mediated toxic factors (e.g. ROS, proinflammatory cytokines and effectors of necroptosis and apoptosis) and the astrocyte secretome as mediators of MN homeostasis needs to be further investigated.
Motor neurons
Although neurons are not key players in the actual inflammation process, alterations in their cellular homeostasis can make them more or less vulnerable to inflammatory stimuli and hence contribute to inflammation-induced MN loss. One such example was recently identified in human induced motor neurons (iMNs) carrying the C9orf72 HRE. In these cells, the reduction of C9orf72 protein levels led to an increase of glutamate receptors on the iMNs membrane causing excitotoxicity in response to extracellular glutamate [63]. As mentioned above, excitotoxicity can further be enhanced by reduced glutamate clearance by astrocytes by loss of EAAT2 glutamate transporters [169, 170]. On top of that, neuronal damage itself may cause the release of self-antigens and proinflammatory factors that activate resting astrocytes and microglia. These will, as mentioned before, produce neurotoxic factors such as NO, ROS, pro-inflammatory cytokines resulting in a viscous feedback-loop that aggravates the neuroinflammation in the CNS [141, 171].
Peripheral immune system
Under normal conditions, the CNS immune system and peripheral immune cells are separated by the BBB and blood-cerebrospinal fluid barrier (B-CSF-B) which limit the entry of circulating toxic molecules and pathogens into the CNS [171]. In case of injury, the peripheral immune system will invade the CNS causing neuroinflammation by infiltration of leukocytes and macrophages [172,173,174].
Monocytes/Macrophages
Monocytes are a central constituent of the peripheral innate immune system which are derived from precursors in the bone marrow. Monocytes can differentiate further into macrophages or dendritic cells (DCs) as they migrate into tissues and participate in the response to an inflammatory stimulus. Macrophages have multiple functional similarities with microglia in the CNS. First, macrophages express TLRs and therefore can be activated by PAMPs, endogenous molecules released by injured tissues and necrotic cells, such as DAMPs and aggregated proteins [175]. Second, they have the capability to activate Th1 cells by presenting antigens through expression of major histocompatibility complex (MHC) class II molecules [176]. Third, by interacting with Th cells and Tregs they can differentiate into either an anti-inflammatory and neuroprotective M2 phenotype or pro-inflammatory and neurotoxic M1 phenotype [177].
Toll-like receptor 4 (TLR4), a transmembrane protein that is involved the recognition of PAMPs and DAMPs is upregulated in peripheral blood mononuclear cells obtained from sALS patients, suggesting a chronic activation of monocytes in ALS [178]. In addition, there are several reports of increased CNS infiltration of monocytes in ALS patients, and of altered CNS infiltration in asymp-C9, both suggesting the possibility that CNS invading monocytes may have a modifying role in the context of ALS pathogenesis [179, 180]. Moreover, elevated levels of proinflammatory molecules including CCL2, IL-1b, IL-8, FosB, C-X-C motif ligand (CXCL)-1 and CXCL2 have been documented in the cerebrospinal fluid (CSF) and spinal cord of ALS patients [109, 179, 181, 182]. There is also evidence for an imbalance between M1 and M2 macrophages in ALS pathophysiology [183, 184]. M1 macrophages in ALS patients have been demonstrated to produce more pro-inflammatory cytokines than M1 macrophages from healthy individuals [185]. Notably, among these pro-inflammatory cytokines, IL-6 and TNFα levels are positively correlated with disease burden and disease progression, respectively. A positive correlation between the disease progression rate and macrophage infiltration and complement secretion in peripheral nerves has also been reported [186,187,188].
Among the myeloid cells, C9orf72 is highly expressed specifically in CD14 + monocytes [189, 190]. Interestingly, Rizuu et. al, showed the presence of RNA foci in CD14 + monocytes in brain tissue of C9-carriers [190]. In C9orf72 -/- mice, the levels of IL-12, IL-17a, IL-10 and TNF in serum were increased compared to wild-type mice [137]. Moreover, RNA sequencing studies from brain and lymphoid tissues revealed perturbations in genes involved in immune responses.
T cells
T cells, originating from lymphoid stem cells in bone marrow progenitors and maturing in the thymus, express T cell receptors (TCR). On their surface, T cells can express either CD8 glycoprotein (cytotoxic CD8 + T cell) or CD4 glycoprotein (CD4 + cells). Furthermore, CD4 + naive cells differentiate into different subsets called T helpers (Th1, 2, 9, 17, 22), Treg, and follicular helper T cells (Tfh). These subset are characterized by different cytokine profiles and are involved in the elimination of intracellular pathogens and in the attenuation of neuroinflammatory processes [191,192,193]. In many neurodegenerative diseases CD4 + T cells infiltrate the CNS where they interact predominantly with microglial cells and promote neuroinflammation ultimately leading to neuronal damage and neurodegeneration [127, 194]. Infiltration of T cells and hyperactivation of resident immune cells has been demonstrated in post-mortem spinal cords of ALS patients [109, 195]. CD4 + cells are detected near the ventral horn and corticospinal tract, implicating their infiltration into the CNS during the disease course [120]. Tregs are a key cell type responsible for suppressing the immune response, thereby maintaining homeostasis and self-tolerance [196]. Tregs have shown to be neuroprotective in ALS and levels of Tregs have been shown to inversely correlate with disease progression rates [183, 197, 198]. CD8 + cells infiltrate in the CNS and have the capability to participate in regulation of the immune response by producing cytokines such as IFN-γ and TNF-α [199, 200]. Unlike CD4 + cells that are involved in different stages of the disease, the CD8 + cells have only been observed in later stages of the disease [198, 200,201,202]. CD8 + cells positively correlate with neuronal function in the peripheral nerves [203]. Increased levels of CD4 + cells, decreased levels of CD8 + cells and Tregs (CD4 + /CD25 +) in peripheral blood were detected in ALS patients compared to healthy individuals [204]. However, to what extent peripheral T cell contribute to ALS pathogenesis is controversial and the question remains whether the level of circulating T cells is of importance to disease-related immune responses in ALS. Increased levels of CD4 + cells and decreased levels of CD8 + cells and Tregs (CD4 + /CD25 +) were detected in ALS patients compared to healthy individuals [204]. Little is known about T cells in C-ALS/FTD patients. Studies in C9orf72 -/- mice revealed some interesting signs of immune system dysregulation [137], such as: 1) significantly increased percentages of CD8 + cells expressing programmed death protein 1, 2) increased CD69 and CD44 (markers of effector memory T cells) levels in spleen and kidney, 3) increased CD44, CD69 and PD-1 expression in CD4 + cells in spleen, kidney and cervical lymph node, 4) increased percentages of Tregs in spleens and cervical lymph nodes, and 5) reduced expression of CD62L and CD127 (markers of the naïve and central memory T cells) in spleen. These kind of studies in C9-ALS/FTD patients are needed to explore the role of T cells and their subpopulations in neurodegeneration in patients.
NK cells
Natural killer cells (NK) are bone marrow-derived hematopoietic cells that are capable of directly attacking and killing target cells through interaction of FasL protein and the Fas receptor which triggers apoptosis through the classical caspase cascade and by the release of cytotoxic granules containing granzyme and perforin [205, 206]. As such, NK and CD8 + T cells are both cytotoxic effector cells of the immune system. Additionally, NK cells are able to produce chemokines and cytokines through stimulation of activatory or inhibitory receptors.
In case of CNS inflammation, peripheral NK cells are recruited to the CNS by chemokines such as CX3C ligand 1 (CX3CL1), CCL2 and CXC ligand 10 (CXCL10), produced by neurons and microglia, astrocytes, and other inflammatory cells, respectively [207, 208].
Although there is some evidence for a role of NK cells in ALS, little is known in the context of C9-ALS/FTD. In the spinal cord of end-stage mSOD1 mice, the presence of NK cells has been demonstrated [201]. In the blood of ALS patients and in post mortem ALS spinal cord and motor cortex, an increased number of NK cells has also been reported [209]. In addition, a CCL2-dependent accumulation of NK cells in the motor cortex and spinal cord of hSOD1G93A and TDP43A315T ALS mice models has been described [210]. These NK cells mediate microglia and astrocyte proinflammatory polarization via secretion of IFNγ and inhibit CNS infiltration of Treg cells. Moreover, since MNs express natural killer group 2D (NK2GD) ligands, NK cells may also directly induce MN death [210]. Interestingly, depletion of NK cells slows down the rate of neurodegeneration and increases the survival in these mice models of ALS, further underscoring the involvement of NK cells in both the onset and progression of MN death in ALS [210]. While the involvement of NK cells in the modulation of neuroinflammation in neurodegenerative disease has been reported [211,212,213], the precise role of NK cells as protective or deleterious factors in ALS and FTD specifically needs to be further explored.
A recent study showed changes in lymphocyte subpopulations including NK cells, CD4 + naïve cells and CD8 + cells subtype, effector memory cells, in C9-ALS patients compared to non-C9-ALS patients [214]. NK and T cells can presumably act as prognostic markers for survival. However, a large cohort study is required for a more in-depth validation.
A special focus on STING
Recently, a new key player in the field of immunity, has been linked to ALS and C9-ALS/FTD [215, 216]. This pathway is called cyclic GMP–AMP synthase (cGAS)–stimulator of interferon genes (STING) pathway as illustrated in Fig. 4. Besides being a crucial component of the innate immune system, STING signaling is also involved in autophagy, apoptosis and necroptosis [217,218,219]. Cytosolic DNA is sensed by a DNA sensor protein cGAS to catalyze cyclic GMP–AMP (cGAMP) by using ATP and GTP as substrates. This generates the second messenger cGAMP which then binds to the ER-localized adaptor protein STING. Translocation from the ER to the Golgi and subsequent phosphorylation occurs. The phosphorylation can also occur through ER-stress by ROS production as a result of cytosolic TDP-43 and DPR protein in the mitochondria. Additionally, TBK1 phosphorylates IRF3, leading to dimerization, nuclear translocation and eventually activation of the transcription of genes encoding type I interferons such as interferon-β (IFNβ). IκB kinase (IKK) phosphorylates NF-κB inhibitor IκBα, resulting in nuclear translocation of NF-κB. This will activate the transcription of genes encoding for proinflammatory cytokines such as IL-6 and TNF and eventually trigger the immune response (Fig. 4). Recently, a link between TDP-43 and the STING pathway has been elucidated [216, 220]. In iPSC-derived MNs from TDP-43 ALS patients and mutant TDP-43 mice, TDP-43 was found to mislocalize to the cytoplasm and enter the mitochondria. This mitochondrial localization of TDP-43 induced ROS production and the release of mitochondrial DNA, which activated the STING pathway [220].
Overview of the STING pathway, the involvement of C9orf72 and its relation to C9 ALS/FTD. Cytosolic (mislocalized) TDP-43 species and/or DPR proteins cause mitochondrial damage and lead to the increased production and release of toxic ROS and mitochondrial DNA (mtDNA). cGAS, which is a cytosolic DNA sensor, binds the mtDNA and catalyzes the production of cGAMP which in turn results in stimulator of interferon genes (STING) protein dimerization. STING dimers are able to translocate to the Golgi, where they bind and activate TANK binding kinase 1 (TBK1). This complex will then phosphorylate inhibitor of NF-κBα (IκBα) and interferon regulatory factor 3 (IRF3) and cause both transcription factors to localize to the nucleus and promote the transcription of proinflammatory cytokines and type I interferons. The active STING-TBK1 signalosome is involved in autophagosome formation, an initial step in the process of autophagy. Interestingly, STING is degraded itself by autophagy and thus has an elegant self-regulatory mechanism to control its levels and the activation of the STING-based immune response. Moreover, as C9orf72 is also involved in endolysosomal trafficking and autophagy, reduced levels of the C9orf72 protein may cause a delay or failure of STING degradation and a hyperactivation of the type I interferon response. ATP: adenosine triphosphate; cGAS: cyclic guanosine monophosphate–adenosine monophosphate (cGAMP) synthase; GTP: guanosine triphosphate; IL-6: interleukin 6; IRF3: interferon regulatory factor 3; mtDNA: mitochondrial DNA; NFκB: nuclear factor-κB; OPTN: optineurin; P: phosphorylation; P62: sequestome 1/ ubiquitin-binding protein p62; STING: stimulator of interferon genes; TBK1: TANK binding kinase 1; TDP-43: TAR DNA-binding protein 43; TNF: tumor necrosis factor
Interestingly, a study has reported an early upregulation of the type I interferon response and adaptive immune activation in DCs isolated from C9orf72 -/- mouse models, suggesting involvement of the STING-induced inflammation in C9orf72 pathology [221,222,223]. The STING signalling pathway is mainly regulated by degradation of the STING protein through autophagy [138]. As the C9orf72 protein is involved in endolysosomal trafficking and autophagy, a loss of C9orf72 levels results in the delayed degradation of the STING protein and an increased activation of the type I interferon response. Moreover, pharmacological inhibition of this pathway by the use of a STING antagonist was able to mitigate the hyperactive type I interferon response, further underscoring the STING pathway as potential therapeutic target [222].
The possible role of the gut microbiome
There is accumulating evidence that gut microbiomes contribute to neurodegenerative diseases [224,225,226]. Differences in gut microbiota have been reported between ALS cases and controls [226]. Nevertheless, some studies found evidence for changes in the gut microbiome, but this could not be confirmed by other studies [227,228,229,230,231,232,233]. Wether these changes are related to dietary changes or truly contributing to neurodegeneration requires further study.
Recently, the role of gut microbiota has been investigated in C9orf72 knockout mouse models [234]. This was studied in 2 colonies including C9orf72Harvard and C9orf72Broad mice. Compared to C9orf72Broad mice, C9orf72Harvard mice had a shorter lifespan, severe movement problems and elevated blood inflammatory markers, such as elevated cytokines and autoimmune antibodies. In the microbiome of C9orf72Harvard mice, murine norovirus, Helicobacter, Pasteurella pneumotropica and Tritrichomonas muris were more commonly detected and a reduced microbiome was found, in comparison with C9orf72Broad. Interestingly, treating the C9orf72Harvard with the transplants of faecal microbiota from C9orf72Broad mice. Treatment with antibiotics could revert this inflammatory phenotype and reduce the infiltration of neutrophils and CD3e + T cells in the spinal cord, highlighting a possible role of gut microbiota-mediated inflammation in the CNS.
Evidence in C9-ALS/FTD patients
Blood studies
A systemic immune response in patients with neurodegenerative brain diseases may, first of all, be measured through elevation of inflammatory cytokines, immune cells and other markers of inflammation in the blood.
Clinical studies comparing blood-based markers of inflammation in C9-ALS/FTD patients are scarce. One group found no differences in plasma MCP-1, ‘regulated upon activation, normal T cell expressed and presumably secreted’ (RANTES), IL-8, or IL-1β, but reported a trend towards increased IL-10 levels in C9-carriers [235]. Another study reported a significantly decreased level of high density lipoprotein (HDL) in FTD patients with a C9orf72 HRE versus FTD patients without such mutation [235]. A decrease in HDL is a marker of systemic inflammation [236]. The levels of very low- and low-density lipoprotein subclasses, high sensitivity C-reactive protein (hs-CRP) or glycoprotein acetyls (GlycA) were not different. A recent study of biomarkers of inflammation in ALS patients and healthy controls revealed an increased plasma TNF‐α level in C9-ALS patients in comparison with non-C9-ALS patients and controls, suggesting a pronounced role of inflammation in C9-ALS [237]. Less is known about changes of blood inflammatory markers in FTD. Some studies reported signs of systemic inflammation in FTD patients, such as increased levels of IL-6 [238].
CSF studies
Several studies have reported changes of inflammatory biomarkers in the CSF from patients with FTD and ALS.
In C9-ALS/FTD patients, relatively few studies of neuroinflammatory CSF biomarkers have been performed. A recent comparison between low numbers of FTD patients with different mutations did no identify increased CSF levels of chitotriosidase (CHIT1) and YKL-40 in C9orf72 HRE patients [239].
A study comparing CSF biomarker profiles in ALS patients with slow and fast disease progression and controls pointed towards more neuroinflammation in C9-ALS [240]. While CSF IL‐15 and soluble triggering receptor expressed on myeloid cells 2 (sTREM2) levels were not elevated in the ALS cohort as a whole, they were elevated in the fast‐progression and the C9-ALS patient groups. In addition, MCP-1 and IL-18 levels were more elevated in CSF from the C9-ALS and the fast‐progressing patients. TNF-ɑ was only elevated in the C9-ALS patients [237]. Another recent study confirmed a prominent inflammatory CSF signature in C9-ALS patients [241]. However, as most C9-ALS patients have a fast disease progression, these changes may be associated with a fast disease progression rather then with the C9 genotype as such.
Another study looked at presymptomatic and symptomatic carriers of C9orf72, SOD1, FUS, TARDBP, NEK1, MAPT and GRN mutations. As expected, a significant increase was found in the levels of CHIT1 and YKL-40 in ALS patients carrying any pathogenic mutation. In FTD patients with pathogenic mutations, YKL-40 levels were also elevated, but no subgroup analysis of C9-ALS/FTD patients was reported [242]. A proteomic study confirmed higher CHIT1 levens in C9-ALS versus C9-FTD [243]. A large multicenter study confirmed increased CSF levels of CHIT1 and YKL-40 in FTD patients compared to controls, but C9-carriers were not analysed seperately [244]. In a recent study, we measured CSF biomarkers of neurodegeneration (NfL & pNfH) and inflammation (CHIT1, YKL-40, MCP-1) and correlated them to survival in ALS patients (34,911,782). A multivariate model revealed that the combination of NfL and YKL-40 best fitted the prediction of survival in ALS patients, but we did not observe significant differences in any of the markers between C9-positive and C9-negative patients.
It remains to be seen to what extent the reported changes in inflammatory biomarkers in C9-ALS/FTD are truly caused by the specific mutation, or rather by variability in phenotypic presentations. It could be that there are underlying differences in inflammatory pathomechanisms at play in C9-ALS/FTD, with potentially a disease-specific role of microglia, but more research is needed to further confirm these differences.
Imaging studies
Several neuroimaging techniques allow to detect and quantify markers of neuroinflammation in vivo. These techniques offer the advantage of measuring markers of inflammation non-invasively in the brain of living subjects.
One such marker of inflammation is the visualization of microglial activation in the CNS through imaging of the 18 kDa translocator protein (TSPO), formerly called peripheral benzodiazepine receptor (PBR) using selective TSPO radioligands. TSPO is a transmembrane cholesterol transporter found primarily in the outer mitochondrial membrane of steroid-synthesizing cells and is thought to be a mediator of cellular metabolic adaptation to various stimuli, including oxidative stress [245]. TSPO is expressed by multiple cell types, including glia and neurons [246]. The protein is suspected to play an important role in inflammatory processes, and overexpression of it is thought to lead to microglial activation [247]. This offered great potential for its use as a visualizer of this mechanism through PET imaging. Over the years, several ligands for TSPO PET imaging have been developed [247,248,249]. TSPO PET visualization has proved valuable as a biomarker of inflammation associated with several neurodegenerative diseases, including ALS. It may be used as an early biomarker of disease activity and potentially as a marker of disease progression [250].
In ALS, several studies have shown increased TSPO ligand binding in the primary and supplementary motor cortices, pons, frontal lobe, temporal lobe and thalamus [251,252,253,254,255,256,257,258]. There seems to be a good correlation between the level of tracer uptake and the severity of the clinical presentation [255, 258]. In FTD patients, increased uptake of TSPO tracers was seen in frontotemporal regions and in the basal ganglia [259, 260]. Only a few C9-carriers were included in these studies, but there was a trend towards C9-FTD patients having higher 11C-PK-11195 (TSPO ligand) binding [259]. This suggests that neuroinflammation may be more pronounced in C9-FTD, but further studies are required to confirm this.
Another potential tracer to visualize neuroinflammation is the membrane P2X purinoceptor 7 (P2X7) receptor (P2X7R), which showed modestly promising results in ALS patients [257]. No C9orf72-specific studies have hitherto been performed.
Post-mortem studies
Markers of neuroinflammation are clearly present in C9-ALS/FTD brain and spinal cord tissues upon post-mortem neuropathological investigation. The most commonly used markers for microglial activation are cluster of differentiation 68 (CD68), human leukocyte antigen-D related protein-R (HLA-DR), and ionised calcium-binding adapter molecule 1 (Iba1) [261].
In brains of C9-FTLD patients, more dystrophic microglia are found in the cortex of affected brain regions in comparison with healthy controls [262]. Both the number and the morphology (ramified vs. amoeboid) of CD68-positive cells in the frontal and temporal gray and white matter are changed in C9orf72 [263]. A greater abundance of CD68-high microglia is also observed in the white matter. Similarly, a higher number of Iba1-positive microglia is observed in the frontal lobe, particularly the white matter, in comparison to controls. Microglia were more often rod-shaped in C9-FTLD, which indicates an intermediate state between ramified and amoeboid morphology. Microglial dystrophy, which may be a result of impaired lysosomal mechanisms, was also more common across all brain regions than it was in control brains. However, this parameter was highly variable between individuals [261].
When comparing FTLD subgroups of carriers of different pathogenic mutations and those without (known) genetic cause, interesting results have been reported. In one study, GRN-FTLD and C9-FTLD brains were compared. It was demonstrated that C9-FTLD was associated with an increase of CD68–positive microglia in the hippocampus. However, superficial cortical layers in the medial frontal gyrus had greater microvacuolation and greater density of ameboid microglia in GRN-FTLD compared to C9-FTLD. We must note, however, that only TDP-type A-FTLD brains were included in this study, which may not be an accurate representation of C9orf72 pathology as type A typically represents but a small fraction of C9orf72 cases [262]. In another study, C9-FTLD brains showed a similar level of microglial activation as non-gene-associated FTLD brains and a lower level than MAPT-FTLD brains [263].
In C9-ALS patients, neuropathologic investigation has shown increased microglial activation in the cervical and lumbar corticospinal tract compared to healthy controls. Intriguingly, a positive correlation between the severity of ALS symptomatology, the lesion load of TDP-43 inclusions and the magnitude of microglial activation was found [264]. The increased microglial reaction using CD68 immunohistochemistry in C9-ALS patients in the pyramidal tract was found to be present at all levels including in the matter underlying the motor cortex, mid-crus cerebri, the medullary pyramids and lateral and anterior corticospinal tracts [265].
When comparing C9-ALS patients with non-C9-ALS patient, one study reported that C9-ALS brains showed more extensive microglial pathology in the white matter of the medulla and the motor cortex (as measured by CD68 and Iba1 immunoreactivity) [265]. Increased CD68 immunoreactivity has also been reported in the body and genu of the corpus callosum of C9-ALS when compared to sporadic ALS [266]. Wheter this inflammatory phenotype is directly linked to the presence of a C9orf72 HRE or is a consequence of more aggressive disease in general remains unclear.
Potential anti-inflammatory therapies
There is a high unmet medical need for disease-modifying treatments for FTD and ALS. Riluzole—a glutamatergic transmission inhibitor—and, edaravone—believed to sequester free radicals, ROS and inhibiting oxidative stress—are currently the only FDA-approved therapeutic options for ALS patients [267, 268]. However, they offer only limited effects on disease progression and survival. Modulation of neuroinflammation is a promising way to alter the disease course, in particular in patients with high levels of neuroinflammation, such as C9-ALS/FTD patients. Although a lot of progress has been made in our understanding of the genetic causes and the molecular pathways involved in ALS, there is still a lack of effective therapeutic options due to the heterogeneity of the disease [269].
Several clinical studies have already attempted to diminish inflammation in ALS patients with drugs such as cyclooxygenase–2 (COX) inhibitors, minocycline, sodium chlorite, interferon-beta and thalidomide. So far, no beneficial effects on ALS progression were observed [270]. Little data are available on C9-ALS/FTD patients and no studies have looked at patients with high levels of neuroinflammation separately. In one study (ClinicalTrials.gov Identifier: NCT01277315), anakinra, a recombinant IL-1 receptor antagonist that blocks IL-1β-mediated inflammatory signaling, was used in 19 ALS patients, of which 4 were known to carry a mutation in C9orf72 gene [271]. The disease progression was measured using ALSFRS-R score and forced expiratory vital capacity as secondary endpoint. IL-6 and TNF-α were also measured as inflammatory biomarkers. However, no correlation between genotype and treatment response was found. Importantly, there was no difference in disease progression, but the IL-6 and TNF-α levels in plasma were also not significantly altered by the treatment. Although IL-1 may serve as a pharmacological inflammatory target for ALS, this study had some limitations such as a small number of patients, not measuring the inflammatory parameters in CSF, and lacking a double-blinded placebo control group.
Nevertheless, C9-ALS patients were shown to have an increased type I interferon signature and a treatment with STING inhibitor suppressed this interferon response which could partially explain the involvement of STING as an underlying mechanism of the enhanced inflammatory profile in C9-ALS patients [222]. Interestingly, small-molecule STING inhibitors showed to decrease the pathology features of autoinflammatory disease in mice [272]. Therefore, different STING inhibitors are proposed as novel, promising therapeutic options once their effectivity and safety in the preclinical development has been demonstrated [273, 274]. There is a surge in the number of trials with anti-inflammatory treatments for ALS/FTD. The current trials specifically for C9-ALS/FTD patients are summarized in Table 1.
Conclusion
In this review paper, we outlined the role of neuroinflammation in the pathogenesis of ALS and FTD caused by C9orf72 HRE. The C9orf72 gene is highly expressed in innate immune cells and multiple studies demonstrated dysfunction of the immune system caused by C9orf72 gene mutations. Further studies are needed to clarify the role of neuroinflammation in the initiation and propagation of the neurodegenerative process in C9-ALS/FTD. The crosstalk between the peripheral and CNS immune system also requires further research, as there are many unanswered questions such as: is there an autoimmune component to C9-ALS/FTD? What is the exact role of the inflammatory resonse in the CNS and along the peripheral motor axons? Can changes in the peripheral immune system predict central neuroinflammation and the prognosis of patients? How is the complex process of neuroinflammation contributing to neurotoxicity?
Human biomarker studies focusing on C9-related inflammation are scarce. More specifically, comparisons between sporadic ALS/FTD, C9-ALS/FTD and ALS/FTD without C9 mutations are largely lacking. Although evidence points to a major role for inflammation in the pathogenesis of C9-ALS/FTD, results of clinical trials with anti-inflammatory and immunosuppressive agents have largely been disappointing to date. Early and precise biomarker-based characterization of patients is important in order to identify the subset of patients that are most likely to benefit from anti-inflammatory therapies. Research into identification of reliable disease biomarkers may also aid in measuring the therapeutic effect of novel therapies. Not only wet biomarkers, but also PET imaging may help to identify microglial activation. Robust pharmacodynamic effects on inflammatory biomarkers should be present in order to maximize the chance of success in efficacy trials. Given the role of C9orf72 in the immune system, there is a rationale for anti-inflammatory therapies in C9-ALS/FTD and a better characterization of the neuroinflammatory resonse will hopefully lead to a targetted therapeutic strategy.
Availability of data and materials
Not applicable.
Abbreviations
- AAD-2004:
-
2-Hydroxy-5-[2-(4-trifluromethylphenyl)-ethylaminobenzoic acid]
- AAV:
-
Adeno-associated virus
- ACM:
-
Astrocyte conditioned medium
- AD:
-
Alzheimer's disease
- ALS:
-
Amyotrophic lateral sclerosis
- ALSFRS:
-
ALS Functional Rating Scale
- ANG:
-
Angiogenin
- ANXA1:
-
Annexin A1
- AP-1:
-
Activator protein 1
- Arg1:
-
Arginase 1
- ASC:
-
Apoptosis associated speck-like protein containing a card
- Asymptomatic C9orf72 HREs carriers:
-
Asymp-C9
- ATAXN2:
-
Ataxin-2
- Aβ:
-
Amyloid beta
- BBB:
-
Blood brain barrier
- BDNF:
-
Brain-derived neurotrophic factor
- bvFTD:
-
Behavioral variant frontotemporal dementia
- B-CSF-B:
-
Blood cerebrospinal fluid barrier
- CCL2:
-
C–C motif chemokine ligand 2
- CCNF:
-
Cyclin F
- CCR2:
-
C–C chemokine receptor type 2
- CD:
-
Cluster of differentiation
- cGAMP:
-
Cyclic GMP–AMP
- cGAS:
-
Cyclic GMP–AMP synthase
- CHCHD10:
-
Coiled-coil-helix-coiled-coil-helix domain containing 10
- CHIT1:
-
Chitotriosidase 1
- CHI3L1/YKL-40:
-
Chitinase-3-like-1 protein
- Chi3L3:
-
Chitinase-3-like-3 protein
- CHMP2b:
-
Chromatin-modifying protein 2b
- CNS:
-
Central nervous system
- COX-2:
-
Cyclo-oxygenase 2
- CSF:
-
Cerebrospinal fluid
- CXCL:
-
C-X-C motif ligand
- CX3CR1:
-
CX3 chemokine receptor 1
- CX3CL1:
-
CX3 chemokine ligand 1
- C9orf72:
-
Chromosome 9 open reading frame 72
- C9-ALS/FTD:
-
C9orf72 HRE-mediated ALS/FTD
- C9orf72 hexanucleotide repeat expansion:
-
C9orf72 HRE
- C9orf72 HREs carriers:
-
C9-carriers
- DAMP:
-
Damage-associated molecular pattern molecule
- DC:
-
Dendritic cell
- DENN:
-
Differentially Expressed in Normal and Neoplasia
- DPR:
-
Dipeptide repeat
- EAAT:
-
Excitatory amino acid transporter
- EDN1:
-
Endothelin 1
- EEA1:
-
Early endosome antigen 1
- EOD:
-
Early onset dementia
- ERK:
-
Extracellular signal-regulated kinase
- EWS:
-
Ewing’s sarcoma protein
- EWSR1:
-
Ewing’s sarcoma protein RNA Binding Protein 1
- fALS:
-
Familial ALS
- FasL:
-
Fas ligand
- FET protein family:
-
FUS, Ewing’s sarcoma protein and TATA-binding protein associated factor 2 N
- fFTD:
-
Familial FTD
- FOXP3:
-
Forkhead box P3 transcription factor
- FTD:
-
Frontotemporal dementia
- FTLD:
-
Frontotemporal lobar degeneration
- FTLD-FET:
-
FTLD associated with FET pathology
- FTLD-FUS:
-
FTLD associated with FUS pathology
- FTLD-TAU:
-
FTLD associated with tau pathology
- FTLD-TDP:
-
FTLD associated with TDP-43 pathology
- FTLD-UPS:
-
FTLD associated with UPS pathology
- FUS:
-
Protein fused in sarcoma
- GA:
-
Glycine-alanine
- GDNF:
-
Glial cell-derived neurotrophic factor
- GDP:
-
Guanosine diphosphate
- GEF:
-
Guanine nucleotide exchange factor
- GFAP:
-
Glial fibrillary acidic protein
- GLE1:
-
GLE1 RNA export mediator
- GlycA:
-
Glycoprotein acetyl
- GP:
-
Glycine-proline
- GR:
-
Glycine-arginine
- GRN:
-
Progranulin
- GTP:
-
Guanosine triphosphate
- G-CSF:
-
Granulocyte colony-stimulating factor
- HDL:
-
High density lipoprotein
- HLA-DR:
-
Human leukocyte antigen-D related protein-R
- HMGB1:
-
High mobility group box 1
- hnRNPA1:
-
Heterogeneous nuclear ribonucleoproteins A1
- hnRNPA2B1:
-
Heterogeneous nuclear ribonucleoproteins A2/B1
- HRE:
-
Hexanucleotide repeat expansion
- hs-CRP:
-
High sensitivity C-reactive protein
- Iba1:
-
Ionised calcium-binding adapter molecule 1
- IFN:
-
Interferon
- IGF-1:
-
Insulin-like growth factor
- IKK:
-
IκB kinase
- IL:
-
Interleukin
- iMN:
-
Induced motor neuron
- iPSC:
-
Induced pluripotent stem cell
- iNOS:
-
Inducable nitric oxide synthase
- IRAK:
-
Interleukin-1 receptor-associated kinase
- IRF:
-
Interferon regulatory factors
- JAK:
-
Janus kinase
- JNK:
-
C-Jun N-terminal kinase
- KIF5A:
-
Kinesin family member 5A
- Lamp1:
-
Lysosomal associated membrane protein 1
- LCD:
-
Low-complexity domain
- LPS:
-
Lipopolysaccharides
- lv-PPA:
-
Logopenic variant primary progressive aphasia
- MAPK:
-
Mitogen-activated protein kinase
- MAPT:
-
Microtubule associated protein tau
- MAP2K:
-
Mitogen-activated protein kinase kinase
- MAP3K:
-
Mitogen-activated protein kinase kinase kinase
- MATR3:
-
Matrin 3
- MCP-1:
-
Monocyte chemoattractant protein-1
- MHC:
-
Major histocompatibility complex
- MIP-1a:
-
Macrophage inflammatory protein 1 alpha
- MN:
-
Motor neuron
- MND:
-
Motor neuron disease
- MRC:
-
Medical research councel
- mRNA:
-
Messenger RNA
- mTOR:
-
Mammalian target of rapamycin hyjuèy
- NADPH:
-
Nicotinamide adenine dinucleotide phosphate
- NEK1:
-
Never In Mitosis Gene A-Related Kinase 1
- nfv-PPA:
-
Non-fluent variant primary progressive aphasia
- NF-κB:
-
Nuclear factor kappa light chain enhancer of activated B cells
- NK:
-
Natural killer cell
- NKG2D:
-
Natural killer group 2D
- NLR:
-
Nucleotide-binding oligomerization domain and leucine-rich repeat-containing receptor
- NLRP3:
-
NOD-like receptor family pyrin domain containing 3
- NOX2:
-
NADPH oxidase 2
- NRF2:
-
Nuclear factor erythroid 2-related factor 2
- Nup:
-
Nucleoporin
- OPTN:
-
Optineurin
- PAMP:
-
Pathogen-associated molecular pattern molecule
- PBMC:
-
Peripheral blood mononuclear cell
- PBR:
-
Peripheral benzodiazepine receptor
- PFN1:
-
Profilin 1
- PNS:
-
Peripheral nervous system
- PPA:
-
Primary progressive aphasia
- PR:
-
Proline-arginine
- pTDP-43:
-
Phosphorylated transactive responseDNA binding protein with Mr 43 kDa
- P2RX7:
-
P2X purinoceptor 7
- p62:
-
Autophagy adaptor protein p62/sequestosome1
- RAN:
-
Repeat-associated non-AUG
- Ran:
-
Ras-related nuclear protein
- RanGAP:
-
RanGTPase-activating protein
- RANTES:
-
Regulated upon activation, normal T cell expressed and presumably secreted
- RCC1:
-
Regulator of chromosome condensation
- ROS:
-
Reactive oxygen species
- sALS:
-
Sporadic ALS
- SETX:
-
Senataxin
- SG:
-
Stress granule
- SMCR8:
-
Smith-Magenis Syndrome Chromosome Region, Candidate 8
- SOD1:
-
Cu/Zn-binding superoxide dismutase
- STAT:
-
Signal transducer and activator of transcription
- STING:
-
Stimulator of interferon genes
- sv-PPA:
-
Semantic variant primary progressive aphasia
- SQSTM1:
-
Sequestosome 1
- S1P:
-
Sphingosine 1‐phosphate
- S100b:
-
S100 beta
- TAB:
-
TGF-beta activated kinase 1 binding protein
- TAF15:
-
TATA-binding protein associated factor 2 N
- TARDBP:
-
Transactive response DNA-binding protein
- TBK1:
-
TANK binding kinase 1
- TCR:
-
T cell receptor
- TDP-43:
-
Transactive response DNA binding protein with Mr 43 kDa
- Teff:
-
Effector T cell
- Tfh:
-
Follicular helper T cell
- TGF-β:
-
Transforming growth factor beta
- Th cell:
-
T helper cell
- TIA1:
-
TIA1 cytotoxic granule associated RNA binding protein
- TLR4:
-
Toll-like receptor 4
- TMEM106B:
-
Transmembrane protein 106B
- TNF-α:
-
Tumor necrosis factor-alpha
- TRAF6:
-
TNF receptor-associated factor 6
- TRAIL:
-
TNF-related apoptosis-inducing ligand
- Treg:
-
Regulatory T-cell
- TREM2:
-
Triggering receptor expressed on myeloid cells 2
- TSPO:
-
18 KDa translocator protein
- TUBA4A:
-
Tubulin alpha 4a
- UBQLN2:
-
Ubiquilin 2
- UPS:
-
Ubiquitin/proteasome system
- VAPB:
-
Vesicle-associated membrane protein-associated protein B and C
- VCP:
-
Valosin containing protein
- VEGF:
-
Vascular endothelial growth factor
- WDR41:
-
WD Repeat domain 41
- Ym1:
-
Chitinase-like protein 3
References
Lomen-Hoerth C, Anderson T, Miller B. The overlap of amyotrophic lateral sclerosis and frontotemporal dementia. Neurology. 2002;59(7):1077–9.
Neumann M, Mackenzie IRA. Review: Neuropathology of non-tau frontotemporal lobar degeneration. Neuropathology and applied neurobiology. 2019;45(1):19–40 2018/10/26 ed.
Saberi S, Stauffer JE, Schulte DJ, Ravits J. Neuropathology of Amyotrophic Lateral Sclerosis and Its Variants. Neurol Clin. 2015;33(4):855–76.
Hardiman O, Al-Chalabi A, Chio A, Corr EM, Logroscino G, Robberecht W, et al. Amyotrophic lateral sclerosis. Nat Rev Dis Primers. 2017;5(3):17071.
Oskarsson B, Gendron TF, Staff NP. Amyotrophic Lateral Sclerosis: An Update for 2018. Mayo Clin Proc. 2018;93(11):1617–28.
Talbott EO, Malek AM, Lacomis D. The epidemiology of amyotrophic lateral sclerosis. Handb Clin Neurol. 2016;138:225–38.
Masrori P, Van Damme P. Amyotrophic lateral sclerosis: a clinical review. Eur J Neurol. 2020;27(10):1918–29.
Gorno-Tempini ML, Hillis AE, Weintraub S, Kertesz A, Mendez M, Cappa SF, et al. Classification of primary progressive aphasia and its variants. Neurology. 2011;76(11):1006–14 2011/02/18 ed.
Rascovsky K, Hodges JR, Knopman D, Mendez MF, Kramer JH, Neuhaus J, et al. Sensitivity of revised diagnostic criteria for the behavioural variant of frontotemporal dementia. Brain. 2011;134(Pt 9):2456–77.
Spinelli EG, Mandelli ML, Miller ZA, Santos-Santos MA, Wilson SM, Agosta F, et al. Typical and Atypical Pathology in Primary Progressive Aphasia Variants. Ann Neurol. 2017;81(3):430–43.
Burrell JR, Kiernan MC, Vucic S, Hodges JR. Motor neuron dysfunction in frontotemporal dementia. Brain : a journal of neurology. 2011;134(Pt 9):2582–94 2011/08/16 ed.
Gordon PH, Delgadillo D, Piquard A, Bruneteau G, Pradat P-F, Salachas F, et al. The range and clinical impact of cognitive impairment in French patients with ALS: a cross-sectional study of neuropsychological test performance. Amyotroph Lateral Scler. 2011;12(5):372–8.
Ringholz GM, Appel SH, Bradshaw M, Cooke NA, Mosnik DM, Schulz PE. Prevalence and patterns of cognitive impairment in sporadic ALS. Neurology. 2005;65(4):586–90.
DeJesus-Hernandez M, Mackenzie IR, Boeve BF, Boxer AL, Baker M, Rutherford NJ, et al. Expanded GGGGCC hexanucleotide repeat in noncoding region of C9ORF72 causes chromosome 9p-linked FTD and ALS. Neuron. 2011;72(2):245–56.
Renton AE, Majounie E, Waite A, Simon-Sanchez J, Rollinson S, Gibbs JR, et al. A hexanucleotide repeat expansion in C9ORF72 is the cause of chromosome 9p21-linked ALS-FTD. Neuron. 2011;72(2):257–68 2011/09/29 ed.
Gijselinck I, Van Langenhove T, van der Zee J, Sleegers K, Philtjens S, Kleinberger G, et al. A C9orf72 promoter repeat expansion in a Flanders-Belgian cohort with disorders of the frontotemporal lobar degeneration-amyotrophic lateral sclerosis spectrum: a gene identification study. Lancet Neurol. 2012;11(1):54–65 2011/12/14 ed.
Amor S, Puentes F, Baker D, van der Valk P. Inflammation in neurodegenerative diseases. Immunology. 2010;129(2):154–69.
Majounie E, Renton AE, Mok K, Dopper EGP, Waite A, Rollinson S, et al. Frequency of the C9orf72 hexanucleotide repeat expansion in patients with amyotrophic lateral sclerosis and frontotemporal dementia: a cross-sectional study. Lancet Neurol. 2012;11(4):323–30.
Murphy NA, Arthur KC, Tienari PJ, Houlden H, Chiò A, Traynor BJ. Age-related penetrance of the C9orf72 repeat expansion. Sci Rep. 2017;7(1):2116.
Majounie E, Renton AE, Mok K, Dopper EG, Waite A, Rollinson S, et al. Frequency of the C9orf72 hexanucleotide repeat expansion in patients with amyotrophic lateral sclerosis and frontotemporal dementia: a cross-sectional study. Lancet Neurol. 2012;11(4):323–30 2012/03/13 ed.
Dedeene L, Van Schoor E, Ospitalieri S, Ronisz A, Weishaupt JH, Otto M, et al. Dipeptide repeat protein and TDP-43 pathology along the hypothalamic-pituitary axis in C9orf72 and non-C9orf72 ALS and FTLD-TDP cases. Acta Neuropathol. 2020;140(5):777–81.
Van Mossevelde S, van der Zee J, Gijselinck I, Sleegers K, De Bleecker J, Sieben A, et al. Clinical Evidence of Disease Anticipation in Families Segregating a C9orf72 Repeat Expansion. JAMA Neurol. 2017;74(4):445–52.
van Blitterswijk M, DeJesus-Hernandez M, Niemantsverdriet E, Murray ME, Heckman MG, Diehl NN, et al. Association between repeat sizes and clinical and pathological characteristics in carriers of C9ORF72 repeat expansions (Xpansize-72): a cross-sectional cohort study. Lancet Neurol. 2013;12(10):978–88.
Fournier C, Barbier M, Camuzat A, Anquetil V, Lattante S, Clot F, et al. Relations between C9orf72 expansion size in blood, age at onset, age at collection and transmission across generations in patients and presymptomatic carriers. Neurobiol Aging. 2019;74:234.e1-234.e8 2018/10/20 ed.
Millecamps S, Boillée S, Le Ber I, Seilhean D, Teyssou E, Giraudeau M, et al. Phenotype difference between ALS patients with expanded repeats in C9ORF72 and patients with mutations in other ALS-related genes. J Med Genet. 2012;49(4):258–63.
Kaivola K, Salmi SJ, Jansson L, Launes J, Hokkanen L, Niemi A-K, et al. Carriership of two copies of C9orf72 hexanucleotide repeat intermediate-length alleles is a risk factor for ALS in the Finnish population. Acta Neuropathol Commun. 2020;8(1):187.
van Blitterswijk M, DeJesus-Hernandez M, Niemantsverdriet E, Murray ME, Heckman MG, Diehl NN, et al. Association between repeat sizes and clinical and pathological characteristics in carriers of C9ORF72 repeat expansions (Xpansize-72): a cross-sectional cohort study. Lancet Neurol. 2013;12(10):978–88 2013/09/10 ed.
Fratta P, Polke JM, Newcombe J, Mizielinska S, Lashley T, Poulter M, et al. Screening a UK amyotrophic lateral sclerosis cohort provides evidence of multiple origins of the C9orf72 expansion. Neurobiol Aging. 2015;36(1):546.e1-7.
Jackson JL, Finch NA, Baker MC, Kachergus JM, DeJesus-Hernandez M, Pereira K, et al. Elevated methylation levels, reduced expression levels, and frequent contractions in a clinical cohort of C9orf72 expansion carriers. Mol Neurodegener. 2020;15(1):7.
Nordin A, Akimoto C, Wuolikainen A, Alstermark H, Jonsson P, Birve A, et al. Extensive size variability of the GGGGCC expansion in C9orf72 in both neuronal and non-neuronal tissues in 18 patients with ALS or FTD. Hum Mol Genet. 2015;24(11):3133–42.
Byrne S, Heverin M, Elamin M, Walsh C, Hardiman O. Intermediate repeat expansion length in C9orf72 may be pathological in amyotrophic lateral sclerosis. Amyotroph Lateral Scler Frontotemporal Degener. 2014;15(1–2):148–50.
Gómez-Tortosa E, Gallego J, Guerrero-López R, Marcos A, Gil-Neciga E, Sainz MJ, et al. C9ORF72 hexanucleotide expansions of 20–22 repeats are associated with frontotemporal deterioration. Neurology. 2013;80(4):366–70.
Nordin A, Akimoto C, Wuolikainen A, Alstermark H, Jonsson P, Birve A, et al. Extensive size variability of the GGGGCC expansion in C9orf72 in both neuronal and non-neuronal tissues in 18 patients with ALS or FTD. Hum Mol Genet. 2015;24(11):3133–42 2015/02/26 ed.
Stewart H, Rutherford NJ, Briemberg H, Krieger C, Cashman N, Fabros M, et al. Clinical and pathological features of amyotrophic lateral sclerosis caused by mutation in the C9ORF72 gene on chromosome 9p. Acta Neuropathol. 2012;123(3):409–17.
Simón-Sánchez J, Dopper EGP, Cohn-Hokke PE, Hukema RK, Nicolaou N, Seelaar H, et al. The clinical and pathological phenotype of C9ORF72 hexanucleotide repeat expansions. Brain. 2012;135(Pt 3):723–35.
Mori K, Arzberger T, Grässer FA, Gijselinck I, May S, Rentzsch K, et al. Bidirectional transcripts of the expanded C9orf72 hexanucleotide repeat are translated into aggregating dipeptide repeat proteins. Acta Neuropathol. 2013;126(6):881–93 2013/10/18 ed.
DeJesus-Hernandez M, Finch NA, Wang X, Gendron TF, Bieniek KF, Heckman MG, et al. In-depth clinico-pathological examination of RNA foci in a large cohort of C9ORF72 expansion carriers. Acta Neuropathol. 2017;134(2):255–69.
Zu T, Liu Y, Bañez-Coronel M, Reid T, Pletnikova O, Lewis J, et al. RAN proteins and RNA foci from antisense transcripts in C9ORF72 ALS and frontotemporal dementia. Proc Natl Acad Sci U S A. 2013;110(51):E4968-4977.
Mizielinska S, Lashley T, Norona FE, Clayton EL, Ridler CE, Fratta P, et al. C9orf72 frontotemporal lobar degeneration is characterised by frequent neuronal sense and antisense RNA foci. Acta Neuropathol. 2013;126(6):845–57.
Haeusler AR, Donnelly CJ, Rothstein JD. The expanding biology of the C9orf72 nucleotide repeat expansion in neurodegenerative disease. Nat Rev Neurosci. 2016;17(6):383–95.
Andrade NS, Ramic M, Esanov R, Liu W, Rybin MJ, Gaidosh G, et al. Dipeptide repeat proteins inhibit homology-directed DNA double strand break repair in C9ORF72 ALS/FTD. Mol Neurodegener. 2020;15(1):13.
Ash PEA, Bieniek KF, Gendron TF, Caulfield T, Lin W-L, Dejesus-Hernandez M, et al. Unconventional translation of C9ORF72 GGGGCC expansion generates insoluble polypeptides specific to c9FTD/ALS. Neuron. 2013;77(4):639–46.
Gendron TF, Bieniek KF, Zhang Y-J, Jansen-West K, Ash PEA, Caulfield T, et al. Antisense transcripts of the expanded C9ORF72 hexanucleotide repeat form nuclear RNA foci and undergo repeat-associated non-ATG translation in c9FTD/ALS. Acta Neuropathol. 2013;126(6):829–44.
Vatsavayai SC, Nana AL, Yokoyama JS, Seeley WW. C9orf72-FTD/ALS pathogenesis: evidence from human neuropathological studies. Acta Neuropathol. 2019;137(1):1–26.
Scotter EL, Chen H-J, Shaw CE. TDP-43 Proteinopathy and ALS: Insights into Disease Mechanisms and Therapeutic Targets. Neurotherapeutics. 2015;12(2):352–63.
Suk TR, Rousseaux MWC. The role of TDP-43 mislocalization in amyotrophic lateral sclerosis. Mol Neurodegener. 2020;15(1):45.
Cook CN, Wu Y, Odeh HM, Gendron TF, Jansen-West K, Del Rosso G, Yue M, Jiang P, Gomes E, Tong J, Daughrity LM, Avendano NM, Castanedes-Casey M, Shao W, Oskarsson B, Tomassy GS, McCampbell A, Rigo F, Dickson DW, Shorter J, Zhang YJ, Petrucelli L. C9orf72 poly(GR) aggregation induces TDP-43 proteinopathy. Sci Transl Med. 2020;12(559):eabb3774. https://doi.org/10.1126/scitranslmed.abb3774.
Bigio EH, Weintraub S, Rademakers R, Baker M, Ahmadian SS, Rademaker A, et al. Frontotemporal lobar degeneration with TDP-43 proteinopathy and chromosome 9p repeat expansion in C9ORF72: clinicopathologic correlation. Neuropathology. 2013;33(2):122–33.
Cooper-Knock J, Bury JJ, Heath PR, Wyles M, Higginbottom A, Gelsthorpe C, et al. C9ORF72 GGGGCC Expanded Repeats Produce Splicing Dysregulation which Correlates with Disease Severity in Amyotrophic Lateral Sclerosis. PLoS One. 2015;10(5):e0127376.
Kraemer BC, Schuck T, Wheeler JM, Robinson LC, Trojanowski JQ, Lee VMY, et al. Loss of murine TDP-43 disrupts motor function and plays an essential role in embryogenesis. Acta Neuropathol. 2010;119(4):409–19.
Sieben A, Van Langenhove T, Engelborghs S, Martin JJ, Boon P, Cras P, et al. The genetics and neuropathology of frontotemporal lobar degeneration. Acta neuropathologica. 2012;124(3):353–72 2012/08/15 ed.
Yang C, Wang H, Qiao T, Yang B, Aliaga L, Qiu L, et al. Partial loss of TDP-43 function causes phenotypes of amyotrophic lateral sclerosis. Proc Natl Acad Sci U S A. 2014;111(12):E1121-1129.
Murray ME, DeJesus-Hernandez M, Rutherford NJ, Baker M, Duara R, Graff-Radford NR, et al. Clinical and neuropathologic heterogeneity of c9FTD/ALS associated with hexanucleotide repeat expansion in C9ORF72. Acta Neuropathol. 2011;122(6):673–90.
Gendron TF, Petrucelli L. Disease Mechanisms of C9ORF72 Repeat Expansions. Cold Spring Harb Perspect Med. 2018;8(4).
Frick P, Sellier C, Mackenzie IRA, Cheng C-Y, Tahraoui-Bories J, Martinat C, et al. Novel antibodies reveal presynaptic localization of C9orf72 protein and reduced protein levels in C9orf72 mutation carriers. Acta Neuropathol Commun. 2018;6(1):72.
Xiao S, MacNair L, McGoldrick P, McKeever PM, McLean JR, Zhang M, et al. Isoform-specific antibodies reveal distinct subcellular localizations of C9orf72 in amyotrophic lateral sclerosis. Ann Neurol. 2015;78(4):568–83.
Beckers J, Tharkeshwar AK, Van Damme P. C9orf72 ALS-FTD: recent evidence for dysregulation of the autophagy-lysosome pathway at multiple levels. Autophagy. 2021;26:1–17.
Braems E, Swinnen B, Van Den Bosch L. C9orf72 loss-of-function: a trivial, stand-alone or additive mechanism in C9 ALS/FTD? Acta Neuropathologica [Internet]. 2020. Available from: https://doi.org/10.1007/s00401-020-02214-x
Balendra R, Isaacs AM. C9orf72-mediated ALS and FTD: multiple pathways to disease. Nat Rev Neurol. 2018;14(9):544–58.
Donnelly CJ, Zhang P-W, Pham JT, Haeusler AR, Mistry NA, Vidensky S, et al. RNA toxicity from the ALS/FTD C9ORF72 expansion is mitigated by antisense intervention. Neuron. 2013;80(2):415–28.
Fumagalli L, Young FL, Boeynaems S, De Decker M, Mehta AR, Swijsen A, et al. C9orf72-derived arginine-containing dipeptide repeats associate with axonal transport machinery and impede microtubule-based motility. Sci Adv. 2021;7(15).
Laflamme C, McKeever PM, Kumar R, Schwartz J, Kolahdouzan M, Chen CX, et al. Implementation of an antibody characterization procedure and application to the major ALS/FTD disease gene C9ORF72. Elife. 2019;15:8.
Shi Y, Lin S, Staats KA, Li Y, Chang W-H, Hung S-T, et al. Haploinsufficiency leads to neurodegeneration in C9ORF72 ALS/FTD human induced motor neurons. Nat Med. 2018;24(3):313–25.
Swinnen B, Robberecht W, Van Den Bosch L. RNA toxicity in non-coding repeat expansion disorders. EMBO J. 2020;39(1):e101112.
Cooper-Knock J, Walsh MJ, Higginbottom A, Robin Highley J, Dickman MJ, Edbauer D, et al. Sequestration of multiple RNA recognition motif-containing proteins by C9orf72 repeat expansions. Brain. 2014;137(Pt 7):2040–51.
DeJesus-Hernandez M, Finch NA, Wang X, Gendron TF, Bieniek KF, Heckman MG, et al. In-depth clinico-pathological examination of RNA foci in a large cohort of C9ORF72 expansion carriers. Acta Neuropathol. 2017;134(2):255–69.
Fratta P, Poulter M, Lashley T, Rohrer J, Polke J, Beck J, et al. Homozygosity for the C9orf72 GGGGCC repeat expansion in frontotemporal dementia. Acta neuropathologica [Internet]. 2013;126. Available from: https://link.springer.com/content/pdf/https://doi.org/10.1007/s00401-013-1147-0.pdf
Haeusler AR, Donnelly CJ, Periz G, Simko EAJ, Shaw PG, Kim M-S, et al. C9orf72 nucleotide repeat structures initiate molecular cascades of disease. Nature. 2014;507(7491):195–200.
Zhou B, Geng Y, Liu C, Miao H, Ren Y, Xu N, et al. Characterizations of distinct parallel and antiparallel G-quadruplexes formed by two-repeat ALS and FTD related GGGGCC sequence. Sci Rep. 2018;8(1):2366.
Reddy K, Zamiri B, Stanley SYR, Macgregor RBJ, Pearson CE. The disease-associated r(GGGGCC)n repeat from the C9orf72 gene forms tract length-dependent uni- and multimolecular RNA G-quadruplex structures. J Biol Chem. 2013;288(14):9860–6.
Vatovec S, Kovanda A, Rogelj B. Unconventional features of C9ORF72 expanded repeat in amyotrophic lateral sclerosis and frontotemporal lobar degeneration. Neurobiol Aging. 2014;35(10):2421.e1-2421.e12.
Walker C, Herranz-Martin S, Karyka E, Liao C, Lewis K, Elsayed W, et al. C9orf72 expansion disrupts ATM-mediated chromosomal break repair. Nat Neurosci. 2017;20(9):1225–35.
Nguyen L, Cleary JD, Ranum LPW. Repeat-Associated Non-ATG Translation: Molecular Mechanisms and Contribution to Neurological Disease. Annu Rev Neurosci. 2019;8(42):227–47.
Schmitz A, Pinheiro Marques J, Oertig I, Maharjan N, Saxena S. Emerging Perspectives on Dipeptide Repeat Proteins in C9ORF72 ALS/FTD. Front Cell Neurosci. 2021;15:637548.
Lin Y, Mori E, Kato M, Xiang S, Wu L, Kwon I, et al. Toxic PR Poly-Dipeptides Encoded by the C9orf72 Repeat Expansion Target LC Domain Polymers. Cell. 2016;167(3):789-802.e12.
White MK, Johnson EM, Khalili K. Multiple roles for Puralpha in cellular and viral regulation. Cell Cycle. 2009;8(3):1–7.
Boeynaems S, Bogaert E, Kovacs D, Konijnenberg A, Timmerman E, Volkov A, et al. Phase Separation of C9orf72 Dipeptide Repeats Perturbs Stress Granule Dynamics. Mol Cell. 2017;65(6):1044-1055.e5.
Lee K-H, Zhang P, Kim HJ, Mitrea DM, Sarkar M, Freibaum BD, et al. C9orf72 Dipeptide Repeats Impair the Assembly, Dynamics, and Function of Membrane-Less Organelles. Cell. 2016;167(3):774-788.e17.
Tao Z, Wang H, Xia Q, Li K, Li K, Jiang X, et al. Nucleolar stress and impaired stress granule formation contribute to C9orf72 RAN translation-induced cytotoxicity. Hum Mol Genet. 2015;24(9):2426–41.
Kim HJ, Taylor JP. Lost in Transportation: Nucleocytoplasmic Transport Defects in ALS and Other Neurodegenerative Diseases. Neuron. 2017;96(2):285–97.
Zhang K, Grima JC, Rothstein JD, Lloyd TE. Nucleocytoplasmic transport in C9orf72-mediated ALS/FTD. Nucleus. 2016;7(2):132–7.
Zhang K, Daigle JG, Cunningham KM, Coyne AN, Ruan K, Grima JC, et al. Stress Granule Assembly Disrupts Nucleocytoplasmic Transport. Cell. 2018;173(4):958-971.e17.
Yin S, Lopez-Gonzalez R, Kunz RC, Gangopadhyay J, Borufka C, Gygi SP, et al. Evidence that C9ORF72 Dipeptide Repeat Proteins Associate with U2 snRNP to Cause Mis-splicing in ALS/FTD Patients. Cell Rep. 2017;19(11):2244–56.
Abo-Rady M, Kalmbach N, Pal A, Schludi C, Janosch A, Richter T, et al. Knocking out C9ORF72 Exacerbates Axonal Trafficking Defects Associated with Hexanucleotide Repeat Expansion and Reduces Levels of Heat Shock Proteins. Stem Cell Rep. 2020;14(3):390–405.
Lopez-Gonzalez R, Lu Y, Gendron TF, Karydas A, Tran H, Yang D, et al. Poly(GR) in C9ORF72-Related ALS/FTD Compromises Mitochondrial Function and Increases Oxidative Stress and DNA Damage in iPSC-Derived Motor Neurons. Neuron. 2016;92(2):383–91.
Zhang Y, Chen K, Sloan SA, Bennett ML, Scholze AR, O’Keeffe S, et al. An RNA-sequencing transcriptome and splicing database of glia, neurons, and vascular cells of the cerebral cortex. J Neurosci. 2014;34(36):11929–47.
Ghoreschi K, Laurence A, O’Shea JJ. Janus kinases in immune cell signaling. Immunol Rev. 2009;228(1):273–87.
Kisseleva T, Bhattacharya S, Braunstein J, Schindler CW. Signaling through the JAK/STAT pathway, recent advances and future challenges. Gene. 2002;285(1–2):1–24.
Liongue C, O’Sullivan LA, Trengove MC, Ward AC. Evolution of JAK-STAT pathway components: mechanisms and role in immune system development. PLoS One. 2012;7(3):e32777.
Dominguez E, Rivat C, Pommier B, Mauborgne A, Pohl M. JAK/STAT3 pathway is activated in spinal cord microglia after peripheral nerve injury and contributes to neuropathic pain development in rat. J Neurochem. 2008;107(1):50–60.
Molet J, Mauborgne A, Diallo M, Armand V, Geny D, Villanueva L, et al. Microglial Janus kinase/signal transduction and activator of transcription 3 pathway activity directly impacts astrocyte and spinal neuron characteristics. J Neurochem. 2016;136(1):133–47.
Przanowski P, Dabrowski M, Ellert-Miklaszewska A, Kloss M, Mieczkowski J, Kaza B, et al. The signal transducers Stat1 and Stat3 and their novel target Jmjd3 drive the expression of inflammatory genes in microglia. J Mol Med (Berl). 2014;92(3):239–54.
Okada S, Nakamura M, Katoh H, Miyao T, Shimazaki T, Ishii K, et al. Conditional ablation of Stat3 or Socs3 discloses a dual role for reactive astrocytes after spinal cord injury. Nat Med. 2006;12(7):829–34.
Tyzack GE, Hall CE, Sibley CR, Cymes T, Forostyak S, Carlino G, et al. A neuroprotective astrocyte state is induced by neuronal signal EphB1 but fails in ALS models. Nat Commun. 2017;8(1):1164.
Anderson MA, Burda JE, Ren Y, Ao Y, O’Shea TM, Kawaguchi R, et al. Astrocyte scar formation aids central nervous system axon regeneration. Nature. 2016;532(7598):195–200.
Falcicchia C, Tozzi F, Arancio O, Watterson DM, Origlia N. Involvement of p38 MAPK in Synaptic Function and Dysfunction. Int J Mol Sci. 2020;21(16).
He J, Zhong W, Zhang M, Zhang R, Hu W. P38 Mitogen-activated Protein Kinase and Parkinson’s Disease. Transl Neurosci. 2018;9:147–53.
Sun J, Nan G. The Mitogen-Activated Protein Kinase (MAPK) Signaling Pathway as a Discovery Target in Stroke. J Mol Neurosci. 2016;59(1):90–8.
Zhu X, Lee H, Raina AK, Perry G, Smith MA. The role of mitogen-activated protein kinase pathways in Alzheimer’s disease. Neurosignals. 2002;11(5):270–81.
Obata T, Brown GE, Yaffe MB. MAP kinase pathways activated by stress: the p38 MAPK pathway. Crit Care Med. 2000;28(4 Suppl):N67-77.
He Y, Hara H, Núñez G. Mechanism and Regulation of NLRP3 Inflammasome Activation. Trends Biochem Sci. 2016;41(12):1012–21.
Zhou K, Shi L, Wang Y, Chen S, Zhang J. Recent Advances of the NLRP3 Inflammasome in Central Nervous System Disorders. J Immunol Res. 2016;2016:9238290.
Kelley N, Jeltema D, Duan Y, He Y. The NLRP3 Inflammasome: An Overview of Mechanisms of Activation and Regulation. Int J Mol Sci. 2019;20(13).
Swanson KV, Deng M, Ting JP-Y. The NLRP3 inflammasome: molecular activation and regulation to therapeutics. Nat Rev Immunol. 2019;19(8):477–89.
Gratwicke J, Jahanshahi M, Foltynie T. Parkinson’s disease dementia: a neural networks perspective. Brain. 2015;138(Pt 6):1454–76.
Heneka MT, Carson MJ, El Khoury J, Landreth GE, Brosseron F, Feinstein DL, et al. Neuroinflammation in Alzheimer’s disease. Lancet Neurol. 2015;14(4):388–405.
McGeer PL, McGeer EG. Inflammatory processes in amyotrophic lateral sclerosis. Muscle Nerve. 2002;26(4):459–70.
Bendotti C, Bao Cutrona M, Cheroni C, Grignaschi G, Lo Coco D, Peviani M, et al. Inter- and intracellular signaling in amyotrophic lateral sclerosis: role of p38 mitogen-activated protein kinase. Neurodegener Dis. 2005;2(3–4):128–34.
Henkel JS, Engelhardt JI, Siklós L, Simpson EP, Kim SH, Pan T, et al. Presence of dendritic cells, MCP-1, and activated microglia/macrophages in amyotrophic lateral sclerosis spinal cord tissue. Ann Neurol. 2004;55(2):221–35.
Neusch C, Bähr M, Schneider-Gold C. Glia cells in amyotrophic lateral sclerosis: new clues to understanding an old disease? Muscle Nerve. 2007;35(6):712–24.
Sargsyan SA, Monk PN, Shaw PJ. Microglia as potential contributors to motor neuron injury in amyotrophic lateral sclerosis. Glia. 2005;51(4):241–53.
Sekine Y, Takeda K, Ichijo H. The ASK1-MAP kinase signaling in ER stress and neurodegenerative diseases. Curr Mol Med. 2006;6(1):87–97.
Lyck L, Santamaria ID, Pakkenberg B, Chemnitz J, Schrøder HD, Finsen B, et al. An empirical analysis of the precision of estimating the numbers of neurons and glia in human neocortex using a fractionator-design with sub-sampling. J Neurosci Methods. 2009;182(2):143–56.
Pelvig DP, Pakkenberg H, Stark AK, Pakkenberg B. Neocortical glial cell numbers in human brains. Neurobiol Aging. 2008;29(11):1754–62.
Baufeld C, O’Loughlin E, Calcagno N, Madore C, Butovsky O. Differential contribution of microglia and monocytes in neurodegenerative diseases. J Neural Transm (Vienna). 2018;125(5):809–26.
Hickman S, Izzy S, Sen P, Morsett L, El Khoury J. Microglia in neurodegeneration. Nat Neurosci. 2018;21(10):1359–69.
Hickman SE, Kingery ND, Ohsumi TK, Borowsky ML, Wang L, Means TK, et al. The microglial sensome revealed by direct RNA sequencing. Nat Neurosci. 2013;16(12):1896–905.
Lawson LJ, Perry VH, Dri P, Gordon S. Heterogeneity in the distribution and morphology of microglia in the normal adult mouse brain. Neuroscience. 1990;39(1):151–70.
Clarke BE, Patani R. The microglial component of amyotrophic lateral sclerosis. Brain. 2020;143(12):3526–39.
Engelhardt JI, Tajti J, Appel SH. Lymphocytic infiltrates in the spinal cord in amyotrophic lateral sclerosis. Arch Neurol. 1993;50(1):30–6.
Glass CK, Saijo K, Winner B, Marchetto MC, Gage FH. Mechanisms underlying inflammation in neurodegeneration. Cell. 2010;140(6):918–34.
Liddelow SA, Barres BA. Reactive Astrocytes: Production, Function, and Therapeutic Potential. Immunity. 2017;46(6):957–67.
Ramesh G, MacLean AG, Philipp MT. Cytokines and chemokines at the crossroads of neuroinflammation, neurodegeneration, and neuropathic pain. Mediators Inflamm. 2013;2013:480739.
Geloso MC, Corvino V, Marchese E, Serrano A, Michetti F, D’Ambrosi N. The Dual Role of Microglia in ALS: Mechanisms and Therapeutic Approaches. Front Aging Neurosci. 2017;9:242.
Hooten KG, Beers DR, Zhao W, Appel SH. Protective and Toxic Neuroinflammation in Amyotrophic Lateral Sclerosis. Neurotherapeutics. 2015;12(2):364–75.
Meeter LH, Kaat LD, Rohrer JD, van Swieten JC. Imaging and fluid biomarkers in frontotemporal dementia. Nat Rev Neurol. 2017;13(7):406–19 2017/06/18 ed.
Robelin L, Gonzalez De Aguilar JL. Blood biomarkers for amyotrophic lateral sclerosis: myth or reality? Biomed Res Int. 2014;2014:525097.
Skaper SD, Giusti P, Facci L. Microglia and mast cells: two tracks on the road to neuroinflammation. FASEB J. 2012;26(8):3103–17.
Masuda T, Sankowski R, Staszewski O, Prinz M. Microglia Heterogeneity in the Single-Cell Era. Cell Rep. 2020;30(5):1271–81.
Ransohoff RM. A polarizing question: do M1 and M2 microglia exist? Nat Neurosci. 2016;19(8):987–91.
Hanisch U-K. Functional diversity of microglia - how heterogeneous are they to begin with? Front Cell Neurosci. 2013;7:65.
Speicher AM, Wiendl H, Meuth SG, Pawlowski M. Generating microglia from human pluripotent stem cells: novel in vitro models for the study of neurodegeneration. Mol Neurodegener. 2019;14(1):46.
Lorenzini I, Alsop E, Levy J, Gittings LM, Rabichow BE, Lall D, et al. Activated iPSC-microglia from C9orf72 ALS/FTD patients exhibit endosomal-lysosomal dysfunction. bioRxiv. 202;2020.09.03.277459.
O’Rourke JG, Bogdanik L, Yáñez A, Lall D, Wolf AJ, Muhammad AKMG, et al. C9orf72 is required for proper macrophage and microglial function in mice. Science. 2016;351(6279):1324–9.
Rostalski H, Leskelä S, Huber N, Katisko K, Cajanus A, Solje E, et al. Astrocytes and Microglia as Potential Contributors to the Pathogenesis of C9orf72 Repeat Expansion-Associated FTLD and ALS. Front Neurosci. 2019;13:486.
Zhao W, Beers DR, Thonhoff JR, Thome AD, Faridar A, Wang J, et al. Immunosuppressive Functions of M2 Macrophages Derived from iPSCs of Patients with ALS and Healthy Controls. iScience. 2020;23(6):101192.
Atanasio A, Decman V, White D, Ramos M, Ikiz B, Lee H-C, et al. C9orf72 ablation causes immune dysregulation characterized by leukocyte expansion, autoantibody production, and glomerulonephropathy in mice. Sci Rep. 2016;16(6):23204.
Burberry A, Suzuki N, Wang J-Y, Moccia R, Mordes DA, Stewart MH, et al. Loss-of-function mutations in the C9ORF72 mouse ortholog cause fatal autoimmune disease. Sci Transl Med. 2016;8(347):347ra93.
Sudria-Lopez E, Koppers M, de Wit M, van der Meer C, Westeneng H-J, Zundel CAC, et al. Full ablation of C9orf72 in mice causes immune system-related pathology and neoplastic events but no motor neuron defects. Acta Neuropathol. 2016;132(1):145–7.
Frakes AE, Ferraiuolo L, Haidet-Phillips AM, Schmelzer L, Braun L, Miranda CJ, et al. Microglia induce motor neuron death via the classical NF-κB pathway in amyotrophic lateral sclerosis. Neuron. 2014;81(5):1009–23.
Zhao W, Beers DR, Bell S, Wang J, Wen S, Baloh RH, et al. TDP-43 activates microglia through NF-κB and NLRP3 inflammasome. Exp Neurol. 2015;273:24–35.
Hao Z, Liu L, Tao Z, Wang R, Ren H, Sun H, et al. Motor dysfunction and neurodegeneration in a C9orf72 mouse line expressing poly-PR. Nat Commun. 2019;10(1):2906.
O’Rourke JG, Bogdanik L, Yáñez A, Lall D, Wolf AJ, Muhammad AKMG, et al. C9orf72 is required for proper macrophage and microglial function in mice. Science. 2016;351(6279):1324–9.
Schludi MH, Becker L, Garrett L, Gendron TF, Zhou Q, Schreiber F, et al. Spinal poly-GA inclusions in a C9orf72 mouse model trigger motor deficits and inflammation without neuron loss. Acta Neuropathol. 2017;134(2):241–54.
Zhang Y, Burberry A, Wang J-Y, Sandoe J, Ghosh S, Udeshi ND, et al. The C9orf72-interacting protein Smcr8 is a negative regulator of autoimmunity and lysosomal exocytosis. Genes Dev. 2018;32(13–14):929–43.
McAlpine W, Sun L, Wang K-W, Liu A, Jain R, San Miguel M, et al. Excessive endosomal TLR signaling causes inflammatory disease in mice with defective SMCR8-WDR41-C9ORF72 complex function. Proc Natl Acad Sci U S A. 2018;115(49):E11523–31.
Chew J, Gendron TF, Prudencio M, Sasaguri H, Zhang Y-J, Castanedes-Casey M, et al. Neurodegeneration. C9ORF72 repeat expansions in mice cause TDP-43 pathology, neuronal loss, and behavioral deficits. Science. 2015;348(6239):1151–4.
Chew J, Cook C, Gendron TF, Jansen-West K, Del Rosso G, Daughrity LM, et al. Aberrant deposition of stress granule-resident proteins linked to C9orf72-associated TDP-43 proteinopathy. Mol Neurodegener. 2019;14(1):9.
LaClair KD, Zhou Q, Michaelsen M, Wefers B, Brill MS, Janjic A, et al. Congenic expression of poly-GA but not poly-PR in mice triggers selective neuron loss and interferon responses found in C9orf72 ALS. Acta Neuropathol. 2020;140(2):121–42.
Bélanger M, Allaman I, Magistretti PJ. Brain energy metabolism: focus on astrocyte-neuron metabolic cooperation. Cell Metab. 2011;14(6):724–38.
Burda JE, Sofroniew MV. Reactive gliosis and the multicellular response to CNS damage and disease. Neuron. 2014;81(2):229–48.
Bush TG, Puvanachandra N, Horner CH, Polito A, Ostenfeld T, Svendsen CN, et al. Leukocyte infiltration, neuronal degeneration, and neurite outgrowth after ablation of scar-forming, reactive astrocytes in adult transgenic mice. Neuron. 1999;23(2):297–308.
Garwood CJ, Ratcliffe LE, Simpson JE, Heath PR, Ince PG, Wharton SB. Review: Astrocytes in Alzheimer’s disease and other age-associated dementias: a supporting player with a central role. Neuropathol Appl Neurobiol. 2017;43(4):281–98.
Eddleston M, Mucke L. Molecular profile of reactive astrocytes–implications for their role in neurologic disease. Neuroscience. 1993;54(1):15–36.
Farina C, Aloisi F, Meinl E. Astrocytes are active players in cerebral innate immunity. Trends Immunol. 2007;28(3):138–45.
Jha MK, Jo M, Kim J-H, Suk K. Microglia-Astrocyte Crosstalk: An Intimate Molecular Conversation. Neuroscientist. 2019;25(3):227–40.
Ziff OJ, Clarke BE, Taha DM, Crerar H, Luscombe NM, Patani R. Meta-analysis of human and mouse ALS astrocytes reveals multi-omic signatures of inflammatory reactive states. Genome Res. 2021;32(1):71–84. https://doi.org/10.1101/gr.275939.121.
Escartin C, Galea E, Lakatos A, O’Callaghan JP, Petzold GC, Serrano-Pozo A, et al. Reactive astrocyte nomenclature, definitions, and future directions. Nat Neurosci. 2021;24(3):312–25.
Madill M, McDonagh K, Ma J, Vajda A, McLoughlin P, O’Brien T, et al. Amyotrophic lateral sclerosis patient iPSC-derived astrocytes impair autophagy via non-cell autonomous mechanisms. Mol Brain. 2017;10(1):22.
Meyer K, Ferraiuolo L, Miranda CJ, Likhite S, McElroy S, Renusch S, et al. Direct conversion of patient fibroblasts demonstrates non-cell autonomous toxicity of astrocytes to motor neurons in familial and sporadic ALS. Proc Natl Acad Sci U S A. 2014;111(2):829–32.
Varcianna A, Myszczynska MA, Castelli LM, O’Neill B, Kim Y, Talbot J, et al. Micro-RNAs secreted through astrocyte-derived extracellular vesicles cause neuronal network degeneration in C9orf72 ALS. EBioMedicine. 2019;40:626–35.
Milani P, Gagliardi S, Cova E, Cereda C. SOD1 Transcriptional and Posttranscriptional Regulation and Its Potential Implications in ALS. Neurol Res Int. 2011;2011:458427.
Fomin V, Richard P, Hoque M, Li C, Gu Z, Fissore-O’Leary M, et al. The C9ORF72 Gene, Implicated in Amyotrophic Lateral Sclerosis and Frontotemporal Dementia, Encodes a Protein That Functions in Control of Endothelin and Glutamate Signaling. Mol Cell Biol. 2018;38(22).
Almad AA, Doreswamy A, Gross SK, Richard J-P, Huo Y, Haughey N, et al. Connexin 43 in astrocytes contributes to motor neuron toxicity in amyotrophic lateral sclerosis. Glia. 2016;64(7):1154–69.
Birger A, Ben-Dor I, Ottolenghi M, Turetsky T, Gil Y, Sweetat S, et al. Human iPSC-derived astrocytes from ALS patients with mutated C9ORF72 show increased oxidative stress and neurotoxicity. EBioMedicine. 2019;50:274–89.
Serio A, Bilican B, Barmada SJ, Ando DM, Zhao C, Siller R, et al. Astrocyte pathology and the absence of non-cell autonomy in an induced pluripotent stem cell model of TDP-43 proteinopathy. Proc Natl Acad Sci U S A. 2013;110(12):4697–702.
Zhao C, Devlin A-C, Chouhan AK, Selvaraj BT, Stavrou M, Burr K, et al. Mutant C9orf72 human iPSC-derived astrocytes cause non-cell autonomous motor neuron pathophysiology. Glia. 2020;68(5):1046–64.
Qian K, Huang H, Peterson A, Hu B, Maragakis NJ, Ming G-L, et al. Sporadic ALS Astrocytes Induce Neuronal Degeneration In Vivo. Stem Cell Rep 2017;8(4):843–55.
Kwon I, Xiang S, Kato M, Wu L, Theodoropoulos P, Wang T, et al. Poly-dipeptides encoded by the C9orf72 repeats bind nucleoli, impede RNA biogenesis, and kill cells. Science. 2014;345(6201):1139–45.
Renton AE, Majounie E, Waite A, Simón-Sánchez J, Rollinson S, Gibbs JR, et al. A hexanucleotide repeat expansion in C9ORF72 is the cause of chromosome 9p21-linked ALS-FTD. Neuron. 2011;72(2):257–68.
Winkler EA, Sengillo JD, Sagare AP, Zhao Z, Ma Q, Zuniga E, et al. Blood-spinal cord barrier disruption contributes to early motor-neuron degeneration in ALS-model mice. Proc Natl Acad Sci U S A. 2014;111(11):E1035-1042.
Bowman GL, Dayon L, Kirkland R, Wojcik J, Peyratout G, Severin IC, et al. Blood-brain barrier breakdown, neuroinflammation, and cognitive decline in older adults. Alzheimers Dement. 2018;14(12):1640–50.
Sweeney MD, Sagare AP, Zlokovic BV. Blood-brain barrier breakdown in Alzheimer disease and other neurodegenerative disorders. Nat Rev Neurol. 2018;14(3):133–50.
Zlokovic BV. The blood-brain barrier in health and chronic neurodegenerative disorders. Neuron. 2008;57(2):178–201.
Zhang X, Mosser DM. Macrophage activation by endogenous danger signals. J Pathol. 2008;214(2):161–78.
Chan LLY, Cheung BKW, Li JCB, Lau ASY. A role for STAT3 and cathepsin S in IL-10 down-regulation of IFN-gamma-induced MHC class II molecule on primary human blood macrophages. J Leukoc Biol. 2010;88(2):303–11.
Martinez FO, Gordon S. The M1 and M2 paradigm of macrophage activation: time for reassessment. F1000Prime Rep. 2014;6:13.
Zhang R, Hadlock KG, Do H, Yu S, Honrada R, Champion S, et al. Gene expression profiling in peripheral blood mononuclear cells from patients with sporadic amyotrophic lateral sclerosis (sALS). J Neuroimmunol. 2011;230(1–2):114–23.
Zhao W, Beers DR, Hooten KG, Sieglaff DH, Zhang A, Kalyana-Sundaram S, et al. Characterization of Gene Expression Phenotype in Amyotrophic Lateral Sclerosis Monocytes. JAMA Neurol. 2017;74(6):677–85.
Zondler L, Müller K, Khalaji S, Bliederhäuser C, Ruf WP, Grozdanov V, et al. Peripheral monocytes are functionally altered and invade the CNS in ALS patients. Acta Neuropathol. 2016;132(3):391–411.
Nagata T, Nagano I, Shiote M, Narai H, Murakami T, Hayashi T, et al. Elevation of MCP-1 and MCP-1/VEGF ratio in cerebrospinal fluid of amyotrophic lateral sclerosis patients. Neurol Res. 2007;29(8):772–6.
Zhang R, Gascon R, Miller RG, Gelinas DF, Mass J, Hadlock K, et al. Evidence for systemic immune system alterations in sporadic amyotrophic lateral sclerosis (sALS). J Neuroimmunol. 2005;159(1–2):215–24.
Beers DR, Henkel JS, Zhao W, Wang J, Huang A, Wen S, et al. Endogenous regulatory T lymphocytes ameliorate amyotrophic lateral sclerosis in mice and correlate with disease progression in patients with amyotrophic lateral sclerosis. Brain. 2011;134(Pt 5):1293–314.
Liao B, Zhao W, Beers DR, Henkel JS, Appel SH. Transformation from a neuroprotective to a neurotoxic microglial phenotype in a mouse model of ALS. Exp Neurol. 2012;237(1):147–52.
Du Y, Zhao W, Thonhoff JR, Wang J, Wen S, Appel SH. Increased activation ability of monocytes from ALS patients. Exp Neurol. 2020;328:113259.
Chiu IM, Phatnani H, Kuligowski M, Tapia JC, Carrasco MA, Zhang M, et al. Activation of innate and humoral immunity in the peripheral nervous system of ALS transgenic mice. Proc Natl Acad Sci U S A. 2009;106(49):20960–5.
Graber DJ, Hickey WF, Harris BT. Progressive changes in microglia and macrophages in spinal cord and peripheral nerve in the transgenic rat model of amyotrophic lateral sclerosis. J Neuroinflammation. 2010;28(7):8.
Kano O, Beers DR, Henkel JS, Appel SH. Peripheral nerve inflammation in ALS mice: cause or consequence. Neurology. 2012;78(11):833–5.
Nataf S, Pays L. Gene co-expression analysis unravels a link between C9orf72 and RNA metabolism in myeloid cells. Acta Neuropathol Commun. 2015;15(3):64.
Rizzu P, Blauwendraat C, Heetveld S, Lynes EM, Castillo-Lizardo M, Dhingra A, et al. C9orf72 is differentially expressed in the central nervous system and myeloid cells and consistently reduced in C9orf72, MAPT and GRN mutation carriers. Acta Neuropathol Commun. 2016;4(1):37.
Baruch K, Ron-Harel N, Gal H, Deczkowska A, Shifrut E, Ndifon W, et al. CNS-specific immunity at the choroid plexus shifts toward destructive Th2 inflammation in brain aging. Proc Natl Acad Sci U S A. 2013;110(6):2264–9.
Marin I, Kipnis J. Learning and memory … and the immune system. Learn Mem. 2013;20(10):601–6.
Raphael I, Nalawade S, Eagar TN, Forsthuber TG. T cell subsets and their signature cytokines in autoimmune and inflammatory diseases. Cytokine. 2015;74(1):5–17.
Lucin KM, Wyss-Coray T. Immune activation in brain aging and neurodegeneration: too much or too little? Neuron. 2009;64(1):110–22.
Troost D, van den Oord JJ, de Jong JM, Swaab DF. Lymphocytic infiltration in the spinal cord of patients with amyotrophic lateral sclerosis. Clin Neuropathol. 1989;8(6):289–94.
Sakaguchi S, Miyara M, Costantino CM, Hafler DA. FOXP3+ regulatory T cells in the human immune system. Nat Rev Immunol. 2010;10(7):490–500.
Henkel JS, Beers DR, Wen S, Rivera AL, Toennis KM, Appel JE, et al. Regulatory T-lymphocytes mediate amyotrophic lateral sclerosis progression and survival. EMBO Mol Med. 2013;5(1):64–79.
Zhao W, Beers DR, Appel SH. Immune-mediated mechanisms in the pathoprogression of amyotrophic lateral sclerosis. J Neuroimmune Pharmacol. 2013;8(4):888–99.
Chiu IM, Morimoto ETA, Goodarzi H, Liao JT, O’Keeffe S, Phatnani HP, et al. A neurodegeneration-specific gene-expression signature of acutely isolated microglia from an amyotrophic lateral sclerosis mouse model. Cell Rep. 2013;4(2):385–401.
Chiu IM, Chen A, Zheng Y, Kosaras B, Tsiftsoglou SA, Vartanian TK, et al. T lymphocytes potentiate endogenous neuroprotective inflammation in a mouse model of ALS. Proc Natl Acad Sci U S A. 2008;105(46):17913–8.
Beers DR, Henkel JS, Zhao W, Wang J, Appel SH. CD4+ T cells support glial neuroprotection, slow disease progression, and modify glial morphology in an animal model of inherited ALS. Proc Natl Acad Sci U S A. 2008;105(40):15558–63.
Beers DR, Henkel JS, Zhao W, Wang J, Huang A, Wen S, et al. Endogenous regulatory T lymphocytes ameliorate amyotrophic lateral sclerosis in mice and correlate with disease progression in patients with amyotrophic lateral sclerosis. Brain. 2011;134(Pt 5):1293–314.
Nardo G, Trolese MC, de Vito G, Cecchi R, Riva N, Dina G, et al. Immune response in peripheral axons delays disease progression in SOD1(G93A) mice. J Neuroinflammation. 2016;13(1):261.
Chen X, Feng W, Huang R, Guo X, Chen Y, Zheng Z, et al. Evidence for peripheral immune activation in amyotrophic lateral sclerosis. J Neurol Sci. 2014;347(1–2):90–5.
Di Santo JP. Natural killer cell developmental pathways: a question of balance. Annu Rev Immunol. 2006;24:257–86.
Topham NJ, Hewitt EW. Natural killer cell cytotoxicity: how do they pull the trigger? Immunology. 2009;128(1):7–15.
Shi F-D, Ljunggren H-G, La Cava A, Van Kaer L. Organ-specific features of natural killer cells. Nat Rev Immunol. 2011;11(10):658–71.
Huang D, Shi F-D, Jung S, Pien GC, Wang J, Salazar-Mather TP, et al. The neuronal chemokine CX3CL1/fractalkine selectively recruits NK cells that modify experimental autoimmune encephalomyelitis within the central nervous system. FASEB J. 2006;20(7):896–905.
Murdock BJ, Zhou T, Kashlan SR, Little RJ, Goutman SA, Feldman EL. Correlation of Peripheral Immunity With Rapid Amyotrophic Lateral Sclerosis Progression. JAMA Neurol. 2017;74(12):1446–54.
Garofalo S, Cocozza G, Porzia A, Inghilleri M, Raspa M, Scavizzi F, et al. Natural killer cells modulate motor neuron-immune cell cross talk in models of Amyotrophic Lateral Sclerosis. Nat Commun. 2020;11(1):1773.
Hertwig L, Hamann I, Romero-Suarez S, Millward JM, Pietrek R, Chanvillard C, et al. CX3CR1-dependent recruitment of mature NK cells into the central nervous system contributes to control autoimmune neuroinflammation. Eur J Immunol. 2016;46(8):1984–96.
Infante-Duarte C, Weber A, Krätzschmar J, Prozorovski T, Pikol S, Hamann I, et al. Frequency of blood CX3CR1-positive natural killer cells correlates with disease activity in multiple sclerosis patients. FASEB J. 2005;19(13):1902–4.
Masera RG, Prolo P, Sartori ML, Staurenghi A, Griot G, Ravizza L, et al. Mental deterioration correlates with response of natural killer (NK) cell activity to physiological modifiers in patients with short history of Alzheimer’s disease. Psychoneuroendocrinology. 2002;27(4):447–61.
Cui C, Ingre C, Yin L, Li X, Andersson J, Seitz C, et al. Correlation between leukocyte phenotypes and prognosis of amyotrophic lateral sclerosis: a longitudinal cohort study. medRxiv. 2021;10(05):21264570.
Ablasser A, Chen ZJ. cGAS in action: Expanding roles in immunity and inflammation. Science. 2019;363(6431).
Van Damme P, Robberecht W. STING-Induced Inflammation - A Novel Therapeutic Target in ALS? N Engl J Med. 2021;384(8):765–7.
Gulen MF, Koch U, Haag SM, Schuler F, Apetoh L, Villunger A, et al. Signalling strength determines proapoptotic functions of STING. Nat Commun. 2017;8(1):427.
Sarhan J, Liu BC, Muendlein HI, Weindel CG, Smirnova I, Tang AY, et al. Constitutive interferon signaling maintains critical threshold of MLKL expression to license necroptosis. Cell Death Differ. 2019;26(2):332–47.
Gui X, Yang H, Li T, Tan X, Shi P, Li M, et al. Autophagy induction via STING trafficking is a primordial function of the cGAS pathway. Nature. 2019;567(7747):262–6.
Yu C-H, Davidson S, Harapas CR, Hilton JB, Mlodzianoski MJ, Laohamonthonkul P, et al. TDP-43 Triggers Mitochondrial DNA Release via mPTP to Activate cGAS/STING in ALS. Cell. 2020;183(3):636-649.e18.
Lee JD, Woodruff TM. TDP-43 Puts the STING in ALS. Trends Neurosci. 2020.
McCauley ME, O’Rourke JG, Yáñez A, Markman JL, Ho R, Wang X, et al. C9orf72 in myeloid cells suppresses STING-induced inflammation. Nature. 2020;585(7823):96–101.
McCauley ME, Baloh RH. Inflammation in ALS/FTD pathogenesis. Acta Neuropathol. 2019;137(5):715–30 2018/11/23 ed.
Boziki MK, Kesidou E, Theotokis P, Mentis A-FA, Karafoulidou E, Melnikov M, et al. Microbiome in Multiple Sclerosis; Where Are We, What We Know and Do Not Know. Brain Sci. 2020;10(4).
Łuc M, Misiak B, Pawłowski M, Stańczykiewicz B, Zabłocka A, Szcześniak D, et al. Gut microbiota in dementia. critical review of novel findings and their potential application. Prog Neuropsychopharmacol Biol Psychiatry. 2021;10(104):110039.
Boddy SL, Giovannelli I, Sassani M, Cooper-Knock J, Snyder MP, Segal E, et al. The gut microbiome: a key player in the complexity of amyotrophic lateral sclerosis (ALS). BMC Med. 2021;19(1):13.
Fang X, Wang X, Yang S, Meng F, Wang X, Wei H, et al. Evaluation of the Microbial Diversity in Amyotrophic Lateral Sclerosis Using High-Throughput Sequencing. Front Microbiol. 2016;7:1479.
Rowin J, Xia Y, Jung B, Sun J. Gut inflammation and dysbiosis in human motor neuron disease. Physiol Rep. 2017;5(18).
Zeng Q, Shen J, Chen K, Zhou J, Liao Q, Lu K, et al. The alteration of gut microbiome and metabolism in amyotrophic lateral sclerosis patients. Sci Rep. 2020;10(1):12998.
Ngo ST, Restuadi R, McCrae AF, Van Eijk RP, Garton F, Henderson RD, et al. Progression and survival of patients with motor neuron disease relative to their fecal microbiota. Amyotroph Lateral Scler Frontotemporal Degener. 2020;21(7–8):549–62.
Brenner D, Hiergeist A, Adis C, Mayer B, Gessner A, Ludolph AC, et al. The fecal microbiome of ALS patients. Neurobiol Aging. 2018;61:132–7.
Zhai C-D, Zheng J-J, An B-C, Huang H-F, Tan Z-C. Intestinal microbiota composition in patients with amyotrophic lateral sclerosis: establishment of bacterial and archaeal communities analyses. Chin Med J (Engl). 2019;132(15):1815–22.
Di Gioia D, Bozzi Cionci N, Baffoni L, Amoruso A, Pane M, Mogna L, et al. A prospective longitudinal study on the microbiota composition in amyotrophic lateral sclerosis. BMC Med. 2020;18(1):153.
Burberry A, Wells MF, Limone F, Couto A, Smith KS, Keaney J, et al. C9orf72 suppresses systemic and neural inflammation induced by gut bacteria. Nature. 2020;582(7810):89–94.
Jääskeläinen O, Solje E, Hall A, Katisko K, Korhonen V, Tiainen M, et al. Low Serum High-Density Lipoprotein Cholesterol Levels Associate with the C9orf72 Repeat Expansion in Frontotemporal Lobar Degeneration Patients. J Alzheimers Dis. 2019;72(1):127–37.
Feingold KR, Grunfeld C. The Effect of Inflammation and Infection on Lipids and Lipoproteins. In: Feingold KR, Anawalt B, Boyce A, Chrousos G, de Herder WW, Dungan K, et al., editors. Endotext [Internet]. South Dartmouth (MA): MDText.com, Inc.; 2000 [cited 2020 Dec 12]. Available from: http://www.ncbi.nlm.nih.gov/books/NBK326741/
Huang F, Zhu Y, Hsiao-Nakamoto J, Tang X, Dugas JC, Moscovitch-Lopatin M, et al. Longitudinal biomarkers in amyotrophic lateral sclerosis. Ann Clin Transl Neurol. 2020;7(7):1103–16.
Bossù P, Salani F, Alberici A, Archetti S, Bellelli G, Galimberti D, et al. Loss of function mutations in the progranulin gene are related to pro-inflammatory cytokine dysregulation in frontotemporal lobar degeneration patients. J Neuroinflammation. 2011;6(8):65.
Woollacott IOC, Nicholas JM, Heller C, Foiani MS, Moore KM, Russell LL, et al. Cerebrospinal Fluid YKL-40 and Chitotriosidase Levels in Frontotemporal Dementia Vary by Clinical, Genetic and Pathological Subtype. Dement Geriatr Cogn Disord. 2020;49(1):56–76.
Cedarbaum JM, Stambler N, Malta E, Fuller C, Hilt D, Thurmond B, et al. The ALSFRS-R: a revised ALS functional rating scale that incorporates assessments of respiratory function. BDNF ALS Study Group (Phase III). J Neurol Sci. 1999;169(1–2):13–21.
Pinilla G, Kumar A, Floaters MK, Pardo CA, Rothstein J, Ilieva H. Increased synthesis of pro-inflammatory cytokines in C9ORF72 patients. Amyotroph Lateral Scler Frontotemporal Degener. 2021;30:1–11.
Oeckl P, Weydt P, Steinacker P, Anderl-Straub S, Nordin F, Volk AE, et al. Different neuroinflammatory profile in amyotrophic lateral sclerosis and frontotemporal dementia is linked to the clinical phase. J Neurol Neurosurg Psychiatry. 2019;90(1):4–10.
Barschke P, Oeckl P, Steinacker P, Al Shweiki MR, Weishaupt JH, Landwehrmeyer GB, et al. Different CSF protein profiles in amyotrophic lateral sclerosis and frontotemporal dementia with <em>C9orf72</em> hexanucleotide repeat expansion. J Neurol Neurosurg Psychiatry. 2020;91(5):503.
Abu-Rumeileh S, Steinacker P, Polischi B, Mammana A, Bartoletti-Stella A, Oeckl P, et al. CSF biomarkers of neuroinflammation in distinct forms and subtypes of neurodegenerative dementia. Alzheimers Res Ther. 2019;12(1):2.
Nutma E, Ceyzériat K, Amor S, Tsartsalis S, Millet P, Owen DR, et al. Cellular sources of TSPO expression in healthy and diseased brain. Eur J Nucl Med Mol Imaging. 2021;49(1):146–63.
Cosenza-Nashat M, Zhao M-L, Suh H-S, Morgan J, Natividad R, Morgello S, et al. Expression of the translocator protein of 18 kDa by microglia, macrophages and astrocytes based on immunohistochemical localization in abnormal human brain. Neuropathol Appl Neurobiol. 2009;35(3):306–28.
Dimitrova-Shumkovska J, Krstanoski L, Veenman L. Diagnostic and Therapeutic Potential of TSPO Studies Regarding Neurodegenerative Diseases, Psychiatric Disorders, Alcohol Use Disorders, Traumatic Brain Injury, and Stroke: An Update. Cells. 2020;9(4).
Best L, Ghadery C, Pavese N, Tai YF, Strafella AP. New and Old TSPO PET Radioligands for Imaging Brain Microglial Activation in Neurodegenerative Disease. Curr Neurol Neurosci Rep. 2019;19(5):24.
Chauveau F, Boutin H, Van Camp N, Dollé F, Tavitian B. Nuclear imaging of neuroinflammation: a comprehensive review of [11C]PK11195 challengers. Eur J Nucl Med Mol Imaging. 2008;35(12):2304–19.
Guilarte TR. TSPO in diverse CNS pathologies and psychiatric disease: A critical review and a way forward. Pharmacol Ther. 2019;194:44–58.
Alshikho MJ, Zürcher NR, Loggia ML, Cernasov P, Chonde DB, Izquierdo Garcia D, et al. Glial activation colocalizes with structural abnormalities in amyotrophic lateral sclerosis. Neurology. 2016;87(24):2554–61.
Alshikho MJ, Zürcher NR, Loggia ML, Cernasov P, Reynolds B, Pijanowski O, et al. Integrated magnetic resonance imaging and [(11) C]-PBR28 positron emission tomographic imaging in amyotrophic lateral sclerosis. Ann Neurol. 2018;83(6):1186–97.
Corcia P, Tauber C, Vercoullie J, Arlicot N, Prunier C, Praline J, et al. Molecular imaging of microglial activation in amyotrophic lateral sclerosis. PLoS One. 2012;7(12):e52941.
Ratai E-M, Alshikho MJ, Zürcher NR, Loggia ML, Cebulla CL, Cernasov P, et al. Integrated imaging of [(11)C]-PBR28 PET, MR diffusion and magnetic resonance spectroscopy (1)H-MRS in amyotrophic lateral sclerosis. Neuroimage Clin. 2018;20:357–64.
Turner MR, Cagnin A, Turkheimer FE, Miller CCJ, Shaw CE, Brooks DJ, et al. Evidence of widespread cerebral microglial activation in amyotrophic lateral sclerosis: an [11C](R)-PK11195 positron emission tomography study. Neurobiol Dis. 2004;15(3):601–9.
Van Weehaeghe D, Babu S, De Vocht J, Zürcher NR, Chew S, Tseng C-EJ, et al. Moving Toward Multicenter Therapeutic Trials in Amyotrophic Lateral Sclerosis: Feasibility of Data Pooling Using Different Translocator Protein PET Radioligands. J Nucl Med. 2020;61(11):1621–7.
Van Weehaeghe D, Van Schoor E, De Vocht J, Koole M, Attili B, Celen S, et al. TSPO Versus P2X7 as a Target for Neuroinflammation: An In Vitro and In Vivo Study. J Nucl Med. 2020;61(4):604–7.
Zürcher NR, Loggia ML, Lawson R, Chonde DB, Izquierdo-Garcia D, Yasek JE, et al. Increased in vivo glial activation in patients with amyotrophic lateral sclerosis: assessed with [(11)C]-PBR28. Neuroimage Clin. 2015;7:409–14.
Bevan-Jones WR, Cope TE, Jones PS, Kaalund SS, Passamonti L, Allinson K, et al. Neuroinflammation and protein aggregation co-localize across the frontotemporal dementia spectrum. Brain. 2020;143(3):1010–26.
Cagnin A, Rossor M, Sampson EL, Mackinnon T, Banati RB. In vivo detection of microglial activation in frontotemporal dementia. Ann Neurol. 2004;56(6):894–7.
Woollacott IOC, Toomey CE, Strand C, Courtney R, Benson BC, Rohrer JD, et al. Microglial burden, activation and dystrophy patterns in frontotemporal lobar degeneration. J Neuroinflammation. 2020;17(1):234.
Sakae N, Roemer SF, Bieniek KF, Murray ME, Baker MC, Kasanuki K, et al. Microglia in frontotemporal lobar degeneration with progranulin or C9ORF72 mutations. Ann Clin Transl Neurol. 2019;6(9):1782–96.
Lant SB, Robinson AC, Thompson JC, Rollinson S, Pickering-Brown S, Snowden JS, et al. Patterns of microglial cell activation in frontotemporal lobar degeneration. Neuropathol Appl Neurobiol. 2014;40(6):686–96.
Brettschneider J, Toledo JB, Van Deerlin VM, Elman L, McCluskey L, Lee VM-Y, et al. Microglial activation correlates with disease progression and upper motor neuron clinical symptoms in amyotrophic lateral sclerosis. PLoS One. 2012;7(6):e39216.
Cooper-Knock J, Hewitt C, Highley JR, Brockington A, Milano A, Man S, et al. Clinico-pathological features in amyotrophic lateral sclerosis with expansions in C9ORF72. Brain. 2012;135(Pt 3):751–64.
Cardenas AM, Sarlls JE, Kwan JY, Bageac D, Gala ZS, Danielian LE, et al. Pathology of callosal damage in ALS: An ex-vivo, 7 T diffusion tensor MRI study. Neuroimage Clin. 2017;15:200–8.
Mitchell JD, O’brien MR, Joshi M. Audit of outcomes in motor neuron disease (MND) patients treated with riluzole. Amyotroph Lateral Scler. 2006;7(2):67–71.
Zhang P, Li W, Li L, Wang N, Li X, Gao M, et al. Treatment with edaravone attenuates ischemic brain injury and inhibits neurogenesis in the subventricular zone of adult rats after focal cerebral ischemia and reperfusion injury. Neuroscience. 2012;10(201):297–306.
Petrov D, Mansfield C, Moussy A, Hermine O. ALS clinical trials review: 20 years of failure are we any closer to registering a new treatment? Front Aging Neurosci. 2017;9:68.
Crisafulli SG, Brajkovic S, Cipolat Mis MS, Parente V, Corti S. Therapeutic Strategies Under Development Targeting Inflammatory Mechanisms in Amyotrophic Lateral Sclerosis. Mol Neurobiol. 2018;55(4):2789–813.
Maier A, Deigendesch N, Müller K, Weishaupt JH, Krannich A, Röhle R, et al. Interleukin-1 Antagonist Anakinra in Amyotrophic Lateral Sclerosis–A Pilot Study. PLoS One. 2015;10(10):e0139684.
Haag SM, Gulen MF, Reymond L, Gibelin A, Abrami L, Decout A, et al. Targeting STING with covalent small-molecule inhibitors. Nature. 2018;559(7713):269–73.
Gautier O, Gitler AD. Neurodegenerative gene’s function is not all about those bases. Nature. 2020;585(7823):34–5.
Sheridan C. Drug developers switch gears to inhibit STING. Nat Biotechnol. 2019;37(3):199–201.
Acknowledgements
Not applicable.
Funding
P.M. has a research Fellowship of the European Academy of Neurology (no award/grant number). JM is a PhD fellow at FWO Vlaanderen (11A2321N). PM, JB and PVD are supported by grants from KU Leuven (C1-C14-17–107), Opening the Future Fund (KU Leuven), the Fund for Scientific Research Flanders (FWO-Flanders), the ALS Liga Belgium, the KU Leuven funds ‘Een Hart voor ALS’, ‘Laeversfonds voor ALS Onderzoek’ and the ‘Valery Perrier Race against ALS Fund’, the Alzheimer Research Foundation (SAO-FRA 2017/023), the Flemish Government initiated Flanders Impulse Program on Networks for Dementia Research (VIND 135043), Flanders Innovation and Enterpreneurship (IWT grants Project MinE and iPSCAF), the Belgian National Lottery, the Latran Foundation, the European Union’s Horizon 2020 research and innovation programme (755094) and the European Union’s ERA-Net for Research Programmes on Rare Diseases (INTEGRALS). PVD holds a senior clinical investigatorship of FWO-Vlaanderen and is supported through the E. von Behring Chair for Neuromuscular and Neurodegenerative Disorders.
Author information
Authors and Affiliations
Contributions
Concept and design: PM. Drafting of the manuscript: PM, JB and HG. Creating of the figures: PM and JB. Critical revision of the manuscript for important intellectual content: PM, JB and PVD. All authors read and approved the final manuscript.
Corresponding author
Ethics declarations
Ethics approval and consent to participate
Not applicable.
Consent for publication
Not applicable.
Competing interests
The authors declare to have no conflicts of interests.
Additional information
Publisher’s Note
Springer Nature remains neutral with regard to jurisdictional claims in published maps and institutional affiliations.
Rights and permissions
Open Access This article is licensed under a Creative Commons Attribution 4.0 International License, which permits use, sharing, adaptation, distribution and reproduction in any medium or format, as long as you give appropriate credit to the original author(s) and the source, provide a link to the Creative Commons licence, and indicate if changes were made. The images or other third party material in this article are included in the article's Creative Commons licence, unless indicated otherwise in a credit line to the material. If material is not included in the article's Creative Commons licence and your intended use is not permitted by statutory regulation or exceeds the permitted use, you will need to obtain permission directly from the copyright holder. To view a copy of this licence, visit http://creativecommons.org/licenses/by/4.0/. The Creative Commons Public Domain Dedication waiver (http://creativecommons.org/publicdomain/zero/1.0/) applies to the data made available in this article, unless otherwise stated in a credit line to the data.
About this article
Cite this article
Masrori, P., Beckers, J., Gossye, H. et al. The role of inflammation in neurodegeneration: novel insights into the role of the immune system in C9orf72 HRE-mediated ALS/FTD. Mol Neurodegeneration 17, 22 (2022). https://doi.org/10.1186/s13024-022-00525-z
Received:
Accepted:
Published:
DOI: https://doi.org/10.1186/s13024-022-00525-z