Abstract
Tributyltin (TBT), an aqueous biocide derived from antifouling paint pollution, is known to have impacted coastal marine ecosystems, and has been reported in the sediment of the Norfolk and Suffolk Broads, a network of rivers and shallow lakes in eastern England. In the marine environment, the 1987 TBT ban resulted in expanded use of alternative biocides, raising the question of whether these products too have impacted the Broads ecosystem and freshwaters in general. We examined the lake sediment record in the Norfolk and Suffolk Broads for contamination by copper (Cu), an active biocide agent, and zinc (Zn), a component of booster biocides, to assess their occurrence and potential for causing environmental harm in freshwater ecosystems. After the introduction of leisure boating, there was a statistically significant difference in Cu enrichment between heavily and lightly boated sites, whereas no such difference existed prior to that time. At the heavily boated sites, the onset of Cu enrichment coincided with a period of rapid increase in leisure boating. Such enrichment has been maintained to the present day, with some evidence of continued increase. We conclude that Cu-based antifouling has measurably contaminated lakes exposed to boating, at concentrations high enough to cause ecological harm. Similar findings can be expected at other boated freshwater ecosystems elsewhere in the world.
Similar content being viewed by others
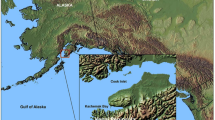
Avoid common mistakes on your manuscript.
Introduction
Marine commercial shipping and leisure craft employ antifouling paints to reduce drag by keeping their hulls clear of biota. The toxic agents contained in these paints were first observed in nature during the 1970s, and since then numerous studies have been undertaken in coastal marine environments (Dahl and Blanck 1996; Voulvoulis et al. 2000; Callow and Callow 2002; Thomas and Brooks 2010; Eklund et al. 2010, 2014; Marcheselli et al. 2010; Parks et al. 2010; Ytreberg et al. 2010; Tsunemasa and Yamazaki 2014), documenting the extent and impact of the contamination. The same agents have also been found in freshwater systems. In the 1980s, Canadian freshwater environments connected with marine shipping were found to have toxic concentrations of TBT (Maguire et al. 1986), and in Lake Lucerne, Switzerland, substantial concentrations of antifouling organotins (including TBT) were found in the water and sediments at marinas (Fent and Hunn 1991). In the Norfolk Broads (East Anglia, eastern England), a network of small, shallow lakes and rivers partially connected with the sea, lake and river sediments were also found to contain TBT in 1989, the levels of which fell substantially by 1992 (Dowson et al. 1994). Despite the significance of these reports, only a few subsequent investigations (Sayer et al. 2006; Lambert et al. 2006; Hoare 2007) considered the extent and impact of environmental pollution by antifouling paints in UK freshwater ecosystems. Sayer et al. (2006) showed that the Norfolk Broads contain a coherent sediment record of the since-banned biocide, TBT. The TBT record is consistent with its historical use, first appearing in the 1960s and then rapidly declining after the 1987 UK ban from vessels <25 m long. The onset of TBT contamination in the Norfolk Broads was coincident with palaeoecological evidence for ecosystem degradation in the form of macrophyte loss and changes in associated invertebrate communities. This, in conjunction with background eutrophication forcing, is suspected to have contributed to the transformation of the lakes from clear-water, macrophyte-dominated to turbid, algal-dominated conditions (Sayer et al. 2006; Holmes et al. 2010).
But what is the effect of other antifouling paint biocides in this freshwater system? TBT was banned in 1987, yet there is no sign of a return to clear-water, macrophyte-dominated conditions in the boated Broads system. This is most likely a consequence of the continued influence of eutrophication, but it is also possible that TBT-replacement antifouling agents are contributing to ecological degradation, and in turn, to poor/slow recovery in the Broads. Indeed there is some evidence consistent with this in the case of the organic antifouling biocide Irgarol 1051 (N-2-methylthio-4-tert-butylamino-6-cyclopropylamino-s-triazine), its metabolite GS26575 (2-methylamino-4-tert-butylamino-6-amino-s-triazine) and diuron (1-(3,4-dichlorophenyl)-3,3-dimethylurea), which were reported by Lambert et al. (2006) to be persistently present in the water of seven broads. The measured environmental concentrations were demonstrated in the laboratory to damage both algal and angiosperm aquatic macrophyte species previously common to the broads surveyed (Lambert et al. 2006). There is still a global market for, and use of these products as booster biocides (i.e. compounds added to an antifoulant to increase efficacy), though since 2001 they have been banned within the UK. Copper is nevertheless widely used as an agent in antifoulants, and a number of organozinc compounds (e.g. zinc pyrithione and zineb) are still in use as booster biocides. Copper is a particular concern in the Broads ecosystems, as correlations have been observed in Hickling Broad between sediment Cu concentrations and measures of photosynthetic stress in the important and now much depleted charophyte community (Smith 2003; Evans 2004). Investigating possible source links, Smith (2003) also analysed interstitial waters in sediments and found that Cu concentrations diminished linearly with distance from the central boating channel. Furthermore, Lambert (2007) found that concentrations of Cu equal to those recorded in the interstitial waters at Hickling Broad in 2005 could be demonstrated to cause a significant reduction in charophyte rhizoid growth when tested under laboratory conditions. These investigations establish a clear link between Cu enrichment and the probability of environmental harm, but do not directly address the source of the Cu contamination.
In this study we tested the possibility that boat antifouling paints are the primary Cu source to the Broads freshwater lakes. That Cu is supplied to the environment by such paints is beyond question: it is an active ingredient, released slowly into the water adjacent to the hull (Dahl and Blanck 1996). It is not, however, straightforward to estimate the relative importance of this pathway in the presence of possible alternative sources. In our study, we examined the sediment records of nine lakes in the Norfolk and Suffolk Broads, assessing temporal changes in the sediment concentrations of Cu (as a biocide agent in its own right), and Zn (as a measure of organo-zinc booster biocides). Four of the lakes are heavily boated and five are either boat-free or only very lightly boated. If boating is a significant source of Cu and Zn, then for sediment deposited since the introduction of leisure boating there should be relative metal enhancement at heavily boated sites. For sediment deposited before that time, any metal enrichment should be independent of boating intensity.
Coring sites and sampling
The Norfolk and Suffolk Broads comprise 60 small (0.01–1.41 km2, median 0.046 km2), shallow (generally <2 m water depth) lakes (termed broads) in eastern England. A 303-km2 part of the area was given National Park status in 1989, and large parts of the area are internationally recognised as the Broadland Ramsar site, and as part of the European Natura 2000 network (The Broads Special Area of Conservation). The broads are flooded medieval peat workings, situated in the valleys of five rivers that converge at the town of Great Yarmouth before discharging into the North Sea (Fig. 1). From the late nineteenth century, the rivers and lakes of the Broads system have formed an important centre for recreational boating (George 1992). Some of the broads are navigable and directly connected to the main river system, whereas others are isolated and are not used by leisure craft. The nine broads included in the current study (Fig. 1) were chosen to represent these two usage categories. Four of the sites are connected to main rivers (Barton Broad, Wroxham Broad, Salhouse Great Broad and Hickling Broad) and are used by boats that employ antifouling paints, whereas five of the sites (Ormesby Great Broad, Ormesby Little Broad, Woolner’s Carr, Sprat’s Water, Barnby Broad) are wholly or partially isolated from the main river system. The broads are alkaline lakes and most, especially the connected broads, are eutrophic and characterised by an absence of submerged macrophytes and prevalence of dense phytoplankton populations (Moss 2001). The sediment in all nine broads comprises a homogeneous mixture of calcareous and organic detritus, with lesser amounts of terrigenous mineral matter, typically with 60–200 cm of sediment lying directly above cut peat surfaces (Ayres et al. 2008; Madgwick et al. 2011).
Materials and methods
Sites
Figure 1 shows the location of the study sites. The heavily boated sites (Barton, Hickling, Salhouse Great and Wroxham Broads) were selected as the largest navigable open water bodies in the upper reaches of three Broadland rivers. The lightly boated sites, all wholly or partially isolated from the navigable rivers, were selected from the Trinity Broads catchment (Ormesby Little and Great Broads) and the Waveney Valley (Barnby Broad, Sprat’s Water and Woolner’s Carr). A single core was collected from each site, from open water locations at least 30–40 m from the lake edge, with evidence for deep-water sediment accumulation.
Sediment coring
Sediment cores were collected using an adapted fat (74-mm i.d.) Livingstone coring system (Livingston 1955), with the exception of the core from Salhouse Great Broad, which was extracted using a wide-bore (140-mm i.d.) Big Ben piston-corer (Patmore et al. 2014). Coring dates, core lengths, sampling intervals, and the chemical analytical methods that were applied are given in Table 1.
Sediment dating
Seven of the nine cores were dated using 210Pb and 137Cs. The radionuclides were measured by gamma assay using Ortec HPGe GWL series well-type germanium detectors at the University of Liverpool (Appleby et al. 1986). Two of the cores were dated using their sediment records of spheroidal carbonaceous particles (SCP). SCP analysis followed Rose (1994, 2008), while dating followed Rose et al. (1995), comparing features in the profiles with the known record. Radiometric dating followed Appleby et al. (1986), by integrating chronological information from bomb testing 137Cs with the decay profiles for naturally occurring unsupported 210Pb. Dating models, and either radionuclide activities or SCP concentrations, are shown in Fig. 2.
Sediment age-depth models for all study lakes. 210Pb (total)—black squares; 137Cs—open circles; spheroidal carbonaceous particles—open diamonds. All 210Pb dating based on CRS model. Thick solid line: age model. Thin solid line: 1 sd uncertainty on age model. Thick dashed line: age model extrapolation based on assumed constant mass accumulation rate
Analytical methods
Metal concentrations were determined by either X-ray fluorescence analysis (XRF) (Boyle 2000) or atomic absorption spectrometry (AAS) on nitric acid extracts. XRF analysis was undertaken using a Bruker S2 Ranger energy dispersive XRF analyser, with calibration and quality control based on a suite of 20 certified reference materials (Table 2).
Standard errors of regression for the reference material (of certified values against measured values, measured as unknowns) for Cu, Pb and Zn were 2.9, 2.7 and 2.2 ppm, respectively. AAS measurements were undertaken using a Unicam 939 Atomic Absorption Spectrophotometer. Sediment was dried at 40 °C. Approximately 0.5 g quantities were weighed accurately and placed in 60-ml high-temperature polythene tubes. 2 ml concentrated AnalR nitric acid was added. When initial reaction had ceased, the tubes were heated at 90 °C for 1 h. When cool, the samples were made up to 24 ml using 10 % HCl. These were centrifuged and decanted. Based on partial extraction from the sediment, AAS-measured concentration values are generally lower than those obtained by XRF (Boyle 2001b). This effect is generally weakest for Pb and strongest for Cu. We have no measurement of the magnitude of the effect in our samples, but expect it does not exceed 20 ppm. Such a difference has little impact on the interpretation of the metal profiles, which depends as much on within-core stratigraphic change as between-core differences.
Organic matter concentration was measured by loss on ignition (LOI). Weight loss was measured after 1 h of ignition at 550 °C on sediment previously dried at 105 °C. Sediment dry density was measured on 1-cm3 subsamples.
We assume that concentrations of Cu, Pb and Zn were not influenced by post-depositional remobilisation, as the mass accumulation rates were sufficient for dissolved fluxes within the sediment to be negligible (Boyle 2001a). We assume that the total metal concentration is made up of the cumulative contributions from natural sources, atmospheric deposition and runoff pollution, and any release from boat antifouling materials. Lastly, we also assume that no analytical procedure can discriminate between these sources, and rely instead on differences in total element concentration between heavily boated and lightly boated sites to infer the relative importance of the different potential sources of metals.
Organotin compounds (tributyltin, dibutyltin and monobutyltin) were analysed by gas chromatography with flame photometric detection, following extraction by sodium hydroxide and methanol, conversion to hydride, and partitioning into hexane (Waldock and Waite 1994). These were measured at only three sites: Wroxham, Hickling and Salhouse Great Broads.
Boating intensity
The sites were divided into two categories, heavily versus lightly boated. No data exist on boat usage for specific sites. Our distinction thus rests on the nature of the sites. Three of the four heavily boated sites, Wroxham Broad, Hickling Broad and Barton Broad have boat yards situated on them, and all are connected to the navigable rivers. The fourth, Salhouse Great Broad, is adjacent to the River Bure and receives very heavy leisure boat traffic. In the lightly boated category, the two Ormesby Broads are used by rowing and sailing boats, which are moored on site. The other three broads have no boating at all, and are fully isolated from the river network.
To measure temporal change in boating intensity we used boat count data (unpublished database, Broads Authority), which are available from 1959 to the present. Data are available for total boat numbers and for total motorboats. We used the latter as most non-motored craft (including small rowing and sailing boats that are stored out of water) do not use antifouling paints, and we assumed that total motorboat number, comprising 69 % of the total boat count (average since 1959) is proportional to the larger craft that do employ such paints. There are no quantitative data on boat numbers for years prior to 1959. Consequently, direct statistical testing of temporal association between boat count and sediment Cu or Zn concentration is not possible. On the other hand, we know that boat count increased rapidly from the start of the record, doubling in the first 8 years and then remaining relatively constant. If we assume that prior to 1959 the boat count was low, then we can statistically test the impact of boats by dividing the record into early (low boat numbers) and late (high boat numbers) intervals. 1960 was chosen as the dividing date as it is mid-way through the period of most rapid boat number increase.
Results
Before describing the sediment records, the significance of strong enrichment of Cu and Zn in surficial sediment must be addressed. Apparent most strikingly at Hickling and Barton Broads, we attribute this to temporary metal storage in the surficial floc layer. Reviewed by Boyle (2000), this commonly observed phenomenon represents only a small quantity of metal, the high concentration reflecting the low dry-matter content of the uppermost sediment rather than high metal contents. These surface enrichments contribute little to the total metal flux (Boyle 2000), and are here considered negligible.
The sediment concentration records of TBT, Cu, Pb and Zn (Fig. 3) reveal a general pattern of early increase and later decrease. In detail, however, the four substances reveal distinctly different temporal trends. The TBT concentration record (measured only at Hickling, Salhouse Great and Wroxham Broads) differs greatly from the metals, being absent prior to ca. 1960. After that date, the concentration builds rapidly to a maximum. High values are maintained until about 1990, after which the concentration declines, abruptly at Salhouse Great Broad and Wroxham Broad, and more gently at Hickling Broad. In contrast to TBT, the metals are present throughout the sediment record. The Pb concentration shows a broad peak in the mid twentieth century, reaching a maximum at approximately 1950, and then declining to the present day. The present day concentrations are ~50 % of the maximum value, and similar in magnitude to the concentrations in sediment dating to 1900. The concentration of Pb is not significantly different between heavily and lightly boated sites, either before or after 1960 (p = 0.34, Table 3). Additionally, the ratio of post-1960 to pre-1960 mean Pb concentration (which provides a simple measure of general enrichment) is not significantly different between heavily and lightly boated sites (Table 4).
Cu and Zn broadly resemble Pb in showing a concentration increase through the first half of the twentieth century, but differ through the late twentieth century in that a concentration decline is weak or absent (Fig. 3). Before 1960 there is no significant difference between the heavily and lightly boated sites with respect to Cu or Zn concentration (Table 3). However, for both Cu and Zn, the post 1960 sediment at heavily boated sites is significantly enriched, on average, relative to lightly boated sites (p = 0.036 and 0.020 for Cu and Zn, respectively [Table 3]). This enrichment is stronger for Cu than for Zn. The ratio of post-1960 over pre-1960 is 1.75 for Cu and 1.47 for Zn. This difference in behaviour between Cu and Zn is seen more strongly in ratios between pre and post-1960 concentrations (Table 4), which reveal a statistically significant increase for Cu only (p = 0.038 for Cu, but 0.231 and 0.380 for Zn and Pb, respectively). To test whether the relative increase in Cu and Zn enrichment coincides with the expansion of leisure boating, the motorboat count record was compared with the Cu/Pb and Zn/Pb ratios (Fig. 4). The Cu/Pb ratio in heavily boated sites shows a distinct stepwise increase in the time interval 1955–1965, coincident with the period of most rapid expansion in leisure motorboats. After this step, the ratio continues to increase. The lightly boated sites do not show a corresponding step, but do show an increase in the later twentieth century in parallel with the records at the heavily boated sites. The Zn/Pb ratio does not show a step-wise increase in any cores; rather the ratio shows a steady increase through the twentieth century at both heavily and lightly boated sites.
Discussion
The sediment geochemical record
The twentieth century sediment record of contamination of these lakes by Cu, Pb, TBT and Zn reveals distinct differences among these contaminants, and in the case of Cu, also a distinct difference between heavily boated and lightly boated sites. Such a difference was also demonstrated by Hoare (2007) for TBT, by comparing lakes connected to and isolated from navigation in the Bure catchment. The pattern of variation can be interpreted in terms of two distinct sources of pollution in addition to any naturally occurring metal, one with a broad mid twentieth century maximum that is independent of boat activity, and another that is associated in both time and space with boat usage.
The sediment Pb enrichment record, characterised by a broad mid-twentieth-century maximum, is seen in all 9 studied lakes and shows no indication of a boat intensity signal. This pattern is typical of lakes across Britain (Foster and Charlesworth 1996; Yang and Rose 2005), and indeed a comparable signal has been reported elsewhere in the developed world (Cochran et al. 1998; Lepland et al. 2010). This is consistent with long-distance transport of atmospheric pollution from mixed urban and industrial sources (Yang and Rose 2005). Between-site differences in the Pb concentration show no systematic geographic pattern, and are likely associated with local factors related to sedimentation rate and focusing. Prior to 1960 this remote source also dominated the supply of Cu and Zn to all nine lakes, and even after 1960 contributed substantially to the sediment records, as seen in the rising Cu/Pb and Zn/Pb ratios at lightly as well as heavily boated sites, consistent with changing atmospheric emissions (National Atmospheric Emissions Inventory, http://naei.defra.gov.uk/overview/ap-overview).
The previously reported association of TBT with the known history of its use in antifouling paints, here strengthened by the addition of new data (the Salhouse Great Broad core), is the most unambiguous evidence of a boating impact on contaminant concentrations. TBT does not occur in nature, and was little used except for the production of antifouling paints, so its origin from boating is certain. This is not the case for Cu, which is used in a wide variety of industries, such that substantial contamination of the sediment took place in the early twentieth century prior to increases in boating intensity. Thus, any signal from antifouling paint contamination must be in the form of an enhancement above previous levels of pollution, and will coexist with the pollution record from continuing alternative sources. Despite these circumstances, which are to a large extent unfavourable for detection of an antifouling paint signal, we find a statistically significant spatial and temporal association of sediment Cu with boating intensity. We find sediment Cu enrichment to occur when two conditions are both met: (1) the lake must have been open to heavy boat traffic, and (2) the sediment must have been deposited during the period of high boating activity. This pattern of spatial and temporal variation is wholly consistent with a boat source for Cu. Although this pattern does not prove a causal link, we have no alternative explanations. For Zn, the situation is less clear. Boat intensity class is positively associated with sediment Zn concentration, but in contrast to Cu, the ratio to Pb does not significantly increase with intensification of boat usage. For this reason we restrict further analysis to Cu.
Procedures exist to distinguish natural from anthropogenic metal in lake sediment records (Hilton et al. 1985; Boyle 2001b; Boes et al. 2011), based on correlations between the metal and natural tracer elements. However, these cannot be applied to Cu at the study sites because none of the potential natural tracer elements show any correlation with Cu in the older sediment. We attribute this to a relatively high exposure of lakes to atmospheric pollution in eastern England, evidenced by a statistically significant correlation of Cu with Pb at all sites for the period prior to 1960 (Table 5). So, instead of applying a natural tracer, we use the past association of Cu with Pb to provide approximate estimates of both the natural and the Pb pollution-related contribution to the sediment total Cu concentration. This is achieved by regressing Cu against Pb. We assume that the intercept provides a measure of the natural component (a slight underestimate because Pb will not naturally fall to zero), and that the regression model provides a measure of Cu associated with Pb pollution. This regression model also provides an estimate of Cu that is neither natural nor related to Pb pollution, a component we refer to as “excess” Cu, and which provides a measure of any boating-related Cu. We quantify post-1960 excess Cu by subtracting regression model Cu (trained on the pre-1960 data) from the measured post-1960 values. Because most of our Cu measurements are based on nitric acid extracts, our natural component neglects any inert mineral-bound Cu. However, two of our sites (Ormesby Little Broad and Salhouse Great Broad) had Cu measured by XRF, which includes mineral-bound Cu. For calculation of percentage natural contributions for these two sites, we substituted the mean natural Cu concentration of the other seven sites. Though not entirely satisfactory, neglecting these two sites does not affect our interpretation. Table 5 shows the percentage Cu contributions calculated in this way, of natural, Pb-pollution related, and “excess” Cu. Though only very approximate, these values suggest that about one-third of the sediment Cu is of natural origin, rather more is related to atmospheric Pb pollution, whereas Cu in excess of these two sources comprises 45 % of the total at the heavily boated sites, and 13 % at the lightly boated sites. We consider it likely that most of the excess Cu at the lightly boated sites is also of atmospheric origin, our estimate being biased by recent reductions in the Cu/Pb ratio of atmospheric pollution (National Atmospheric Emissions Inventory, http://naei.defra.gov.uk/overview/ap-overview).
Environmental significance
As discussed in the preceding section, our data analysis demonstrates that sediment Cu enrichment has taken place at the heavily boated broads. And, although this does not strictly prove a causal association, it is reasonable to suppose that a substantial proportion of the Cu originates from boat antifouling paint. We may also reasonably infer a disproportionate contribution of this boating component to Cu toxicity; whereas the other sources of Cu are active throughout the year, the antifouling paint contribution is intentionally maximal during the plant growing season, which coincides with minimum river discharge and thus maximum aqueous concentrations. We also have evidence from other studies that Cu contamination is associated with loss of charophyte species from UK lakes (Lambert and Davy 2011), and that in marine environments antifouling paints can release Cu at a rate sufficient to cause harm (Ytreberg et al. 2010). What we cannot do, with the information available from the lake sediment record, is directly calculate ecosystem exposure to Cu. With reliable hydrological data for the lakes, and assuming that our single core sites provide unbiased estimates of the lake-wide average sediment Cu burial rate, we could calculate for each site the annual mean total water column Cu concentration. Unfortunately, reliable hydrological data are not generally available, so we can do no more than illustrate, for Hickling Broad, the approximate magnitudes. Hickling Broad has a surface area of 1.3 km2 and hydrological catchment of approximately 16 km2. Assuming a mean annual depth-equivalent runoff rate of 0.21 m yr−1 (Average for local Rivers Ant, Bure, Tud, Wensum and Yare, data from National River Flow Archive, CEH, UK), then the areal water load (total annual water through-put normalised to lake bed area) at Hickling Broad is 2.6 m3 m−2 yr−1. Given a post-1960 sediment Cu burial flux of 11 mg m−2 yr−1, the mean annual total Cu concentration is thus 4.2 µg L−1. This estimate must be regarded as speculative, but does precisely match concentration values recorded in the field in 2005 (Lambert 2007). However, without a means for deciding the temporal distribution of Cu concentration through the year, there is no reliable basis for applying a more sophisticated metal toxicity model. Nonetheless, the magnitude is sufficient to suggest that environmental harm is likely, falling as it does within a range of Cu concentrations demonstrated to be harmful (Brix et al. 2005; Van Sprang et al. 2008). For freshwater systems this is particularly critical, as in aqueous media plants are directly exposed to the harmful effects of Cu and, thus, algae and some species of aquatic higher plants are more easily subjected to Cu toxicity (Gupta 1986; Jana and Choudhuri 1982; Fernandes and Henriques 1991). Carbohydrate synthesis was inhibited in the cyanobacterium Anacystis nidulans exposed to only 0.06 ppm Cu (Gupta 1986), which is of the same order of magnitude predicted to be in the waters of Hickling Broad by this current study and recorded by Lambert in Hickling Broad in August 2005 (Lambert 2007). In further experiments Havens (1994) demonstrated that Cu at concentrations similar to those recorded by Lambert (2007) in the interstitial waters of Hickling Broad in 2005 (100–200 µg l−1) reduced the biomass of zooplankton, ciliates, flagellates, and autotrophic phytoplankton. Bacterial biomass was increased by an order of magnitude relative to the controls.
It is thus evident that substantial ecological impacts may occur at Cu concentrations comparable to those arising from antifouling Cu contamination. So, although we have no direct evidence regarding the ecological impact of the antifouling paint in the Broads system, the indirect evidence for this, combined with the observation that sediment Cu enrichment at boated sites is demonstrable despite high background levels in the area, makes it likely that Cu from antifouling paints is causing harm. Legislation was put in place in 1987 to ban the use of TBT in antifouling paints for small boats (<25 m), and in 2001 further legislative measures were introduced in the UK to restrict usage of “booster biocide” antifouling agents in small (<25 m) vessel paints to dichlofluanid, zinc pyrithione and zineb (Cresswell et al. 2006), effectively banning the use of Irgarol 1051 and diuron. However, the use of Cu as an antifouling substitute for TBT remains unregulated and widely practiced. Our finding of a strong association of Cu with boating, with Cu at levels consistent with environmental harm, suggests a high risk that current legislation is not providing adequate protection to freshwater ecosystems such as the Broads and other inland lakes and wetland systems.
Conclusions
The TBT sediment record for Salhouse Great Broad shows a rapid concentration increase after ca. 1960, followed by a decline ca. 1990, the same temporal signal as previously reported for Hickling Broad and Wroxham Broad. This further supports the interpretation that TBT from boat antifouling paints rapidly contaminated waterways that are connected to the river system, the contamination only declining following the TBT ban in 1987. Pb, a metal not associated with antifouling paints, peaks in the early to mid twentieth century, and is no more enriched at heavily boated than at lightly boated sites. This is consistent with a dominant atmospheric pathway, sourced from remote urban and industrial areas. Cu and Zn show similar behaviour to Pb prior to 1960; the concentrations increase with time and show no difference between lightly and heavily boated sites. However, for the sediment deposited after 1960, the average concentrations are significantly greater in the heavily boated broads. In the case of within-site temporal variations, Cu shows enhancement after the increase in boating intensity, but Zn does not. The Cu/Pb ratio most clearly reveals the stepped character of this Cu increase, the step coinciding in time with a rapid increase in motorboat use in the region between 1955 and 1965. The observed metal concentration data are consistent with two distinct pollution sources. First, Pb with some Zn and Cu is derived via an atmospheric pathway, peaking during the mid twentieth century and declining to the present day. This component changes in composition through the record, the Cu/Pb and Zn/Pb ratios increasing through the last 50 years. Second, TBT and Cu are derived from a local source associated with levels of motorboat activity. This component appears after ca. 1955, and rises rapidly before stabilising after 1970. The TBT contamination declines after 1990, whereas Cu either maintains its level or increases. Zn does not show the same strength of association with boating; if a boating signal is indeed present, then it is effectively masked by alternative sources of Zn.
We consider it likely that present day exposure of the Broads ecosystem to antifouling paint Cu is sufficient to cause ecological harm. If so, then current regulations that permit the use of Cu in antifouling paints do not provide adequate protection to the Broads ecosystem and may hinder ecological recovery. The same may be expected at other boated freshwater ecosystems around the world.
References
Appleby PG, Nolan PJ, Gifford DW, Godfrey MJ, Oldfield F, Anderson NJ, Battarbee RW (1986) 210Pb dating by low background gamma counting. Hydrobiologia 143:21–27
Ayres K, Sayer CD, Perrow M, Skeate E (2008) The contribution of palaeolimnology to shallow lake management: an example from Upton Great Broad, Norfolk, UK. Biodivers Conserv 17:2153–2168
Boes X, Rydberg J, Martinez-Cortizas A, Bindler R, Renberg I (2011) Evaluation of conservative lithogenic elements (Ti, Zr, Al, and Rb) to study anthropogenic element enrichments in lake sediments. J Paleolimnol 46:75–87
Boyle JF (2000) Rapid elemental analysis of sediment samples by isotope source XRF. J Paleolimnol 23:213–221
Boyle J (2001a) Redox remobilization and the heavy metal record in lake sediments: a modelling approach. J Paleolimnol 26:423–431
Boyle JF (2001b) Inorganic geochemical methods in palaeolimnology. In: Last WM, Smol JP (eds) Tracking environmental change using lake sediments. Volume 2: physical and geochemical methods. Kluwer, Dordrecht, pp 83–141
Brix KV, DeForest DK, Burger M, Adams WJ (2005) Assessing the relative sensitivity of aquatic organisms to divalent metals and their representation in toxicity datasets compared to natural aquatic communities. Hum Ecol Risk Assess 11:1139–1156
Callow ME, Callow JA (2002) Marine biofouling: a sticky problem. Biologist 49:10–14
Cochran JK, Hirschberg DJ, Wang J, Dere C (1998) Atmospheric deposition of metals to coastal waters (Long Island Sound, New York U.S.A.): evidence from saltmarsh deposits. Estuar Coast Shelf Sci 46:503–522
Cresswell T, Richards JP, Glegg GA, Readman JW (2006) The impact of legislation on the usage and environmental concentrations of Irgarol 1051 in UK coastal waters. Mar Pollut Bull 52:1169–1175
Dahl B, Blanck H (1996) Toxic effects of the antifouling agent Irgarol 1051 on periphyton communities in coastal water microcosms. Mar Pollut Bull 32:342–350
Dowson PH, Bubb JM, Lester JN (1994) The effectiveness of the 1987 retail ban on TBT based antifouling paints in reducing butyltin concentrations in East Anglia, UK. Chemosphere 28:905–910
Eklund B, Elfström M, Gallego I, Bengtsson BE, Breitholtz M (2010) Biological and chemical characterization of harbour sediments from the Stockholm area. J Soils Sediment 10:127–141
Eklund B, Johansson L, Ytreberg E (2014) Contamination of a boatyard for maintenance of pleasure boats. J Soils Sediment 14:955–967. doi:10.1007/s11368-013-0828-6
Evans LC (2004) Factors affecting the distribution and abundance of the submerged macrophyte community in Hickling Broad, Norfolk: is boating activity having an adverse impact? MSc Thesis, University College London
Fent K, Hunn J (1991) Phenyltins in water, sediment, and biota of freshwater marinas. Environ Sci Technol 25:956–963
Fernandes JC, Henriques FS (1991) Biochemical, physiological, and structural effects of excess copper in plants. Bot Rev 57:246–273
Foster IDL, Charlesworth SM (1996) Heavy metals in the hydrological cycle: trends and explanation. Hydrol Process 10:227–261
George M (1992) The land use, ecology and conservation of Broadland. Packard Publishing Limited, UK
Gupta SL (1986) Copper uptake and inhibition of growth, photosynthetic pigments and macromolecules in the cyanobacterium Anacystis nidulans. Photosynthetica 20:447–453
Havens KE (1994) Structural and functional responses of a freshwater plankton community to acute copper stress. Environ Pollut 86:259–266
Hilton J, Davison W, Ochsenbein U (1985) A mathematical model for analysis of sediment core data—implications for enrichment factor calculations and trace-metal transport mechanisms. Chem Geol 48:281–291
Hoare, DJ (2007) Ecological change in shallow lakes through antifoulant biocide contamination. PhD Thesis, University of London
Holmes J, Sayer CD, Liptrott E, Hoare DJ (2010) Complex controls on ostracod faunal palaeoecology in a shallow, coastal brackish-water lake: implications for palaeosalinity reconstruction. Freshw Biol 55:2484–2498
Jana S, Choudhuri MA (1982) Senescence in submerged aquatic angiosperms—effects of heavy metals. New Phytol 90:477–484
Lambert SJ (2007) The ecological tolerance limits of British (UK) stoneworts (Charophytes). PhD Thesis, University of East Anglia, Norwich
Lambert SJ, Davy AJ (2011) Water quality as a threat to aquatic plants: discriminating between the effects of nitrate, phosphate, boron and heavy metals on charophytes. New Phytol 189:1051–1059
Lambert SJ, Thomas KV, Davy AJ (2006) Assessment of the risk posed by the antifouling booster biocides Irgarol 1051 and diuron to freshwater macrophytes. Chemosphere 63:734–743
Lepland A, Andersen TJ, Lepland A, Arp HPH, Alve E, Breedveld GD, Rindby A (2010) Sedimentation and chronology of heavy metal pollution in Oslo harbor, Norway. Mar Pollut Bull 60:1512–1522
Livingston DA (1955) A lightweight piston sampler for lake deposits. Ecology 36:137–139
Madgwick G, Emson D, Sayer CD, Willby NJ, Rose N, Jackson MJ, Kelly A (2011) Centennial-scale changes to the aquatic vegetation structure of a shallow eutrophic lake and implications for restoration. Freshw Biol 56:2620–2636
Maguire RJ, Tkacz RJ, Chau YK, Bengert GA, Wong PTS (1986) Occurrence of organotin compounds in water and sediment in Canada. Chemosphere 15:253–274
Marcheselli M, Conzo F, Mauri M, Simonini R (2010) Novel antifouling agent-Zinc pyrithione: short- and long-term effects on survival and reproduction of the marine polychaete Dinophilus gyrociliatus. Aquat Toxicol 98:204–210
Moss B (2001) The Broads. Collins, London
Parks R, Donnier-Marechal M, Frickers PE, Turner A, Readman JW (2010) Antifouling biocides in discarded marine paint particles. Mar Pollut Bull 60:1226–1230
Patmore IR, Sayer CD, Goldsmith B, Davidson TA, Rawcliffe R, Salgado J (2014) Big Ben: a new wide bore piston corer for multi-proxy paleolimnology. J Paleolimnol 51:79–86
Rose NL (1994) A note on further refinements to a procedure for the extraction of carbonaceous fly-ash particles from sediments. J Paleolimnol 11:201–204
Rose NL (2008) Quality control in the analysis of lake sediments for spheroidal carbonaceous particles. Limnol Oceanogr Methods 6:172–179
Rose NL, Harlock S, Appleby PG, Battarbee RW (1995) Dating of recent lake sediments in the United Kingdom and Ireland using spheroidal carbonaceous particle (SCP) concentration profiles. Holocene 5:328–335
Sayer CD, Hoare DJ, Simpson GL, Henderson ACG, Liptrot ER, Jackson MJ, Appleby PG, Boyle JF, Jones JI, Waldock MJ (2006) TBT causes regime shift in shallow lakes. Environ Sci Technol 40:5269–5275
Smith DC (2003) The population ecology of charophytes in the context of shallow lake restoration. PhD Thesis, University of East Anglia, Norwich
Thomas KV, Brooks S (2010) The environmental fate and effects of antifouling paint biocides. Biofouling 26:73–88
Tsunemasa N, Yamazaki H (2014) Concentration of antifouling biocides and metals in sediment core samples in the northern part of Hiroshima Bay. Int J Mol Sci 15:9991–10004
Van Sprang P, Vangheluwe M, Van Hyfte A, Heijerick M, Vandenbroele M, Verdonck F (2008) Chapter 3.2 environmental effects. In: Voluntary risk assessment of copper, copper II sulphate pentahydrate, copper (I) oxide, copper (II) oxide, dicopper chloride trihydroxide. European Union Risk Assessment Report, European Chemicals Agency, 179 pp
Voulvoulis N, Scrimshaw MD, Lester JN (2000) Occurrence of four biocides utilized in antifouling paints, as alternatives to organotin compounds, in waters and sediments of a commercial estuary in the UK. Mar Pollut Bull 40:938–946
Waldock MJ, Waite ME (1994) The performance of an analytical method for determination of TBT during a 6-year monitoring program. Appl Organomet Chem 8:649–658
Yang H, Rose N (2005) Trace element pollution records in some UK lake sediments, their history, influence factors and regional differences. Environ Int 31:63–75
Ytreberg E, Karlsson J, Eklund B (2010) Comparison of toxicity and release rates of Cu and Zn from anti-fouling paints leached in natural and artificial brackish seawater. Sci Total Environ 408:2459–2466
Acknowledgments
We thank Andrea Kelly and the Broads Authority for funds to support metals and dating analysis of most of the sediment cores. The Suffolk Broads research was supported by English Nature (now Natural England), Contract No. NB/T/806/02-04.445. The dating and TBT analysis of Salhouse Great Broad was funded from the University of London Central Research Fund (ref AR/ATF/B).
Author information
Authors and Affiliations
Corresponding author
Rights and permissions
Open Access This article is distributed under the terms of the Creative Commons Attribution 4.0 International License (http://creativecommons.org/licenses/by/4.0/), which permits unrestricted use, distribution, and reproduction in any medium, provided you give appropriate credit to the original author(s) and the source, provide a link to the Creative Commons license, and indicate if changes were made.
About this article
Cite this article
Boyle, J.F., Sayer, C.D., Hoare, D. et al. Toxic metal enrichment and boating intensity: sediment records of antifoulant copper in shallow lakes of eastern England. J Paleolimnol 55, 195–208 (2016). https://doi.org/10.1007/s10933-015-9865-z
Received:
Accepted:
Published:
Issue Date:
DOI: https://doi.org/10.1007/s10933-015-9865-z