Abstract
The present study focuses on characterizing the mechanical properties of as-cast and heat-treated 2nd and 3rd generation intermetallic TiAl alloys, which is of practical importance considering its application in the aerospace and automobile industries. Assessing the small-scale properties is crucial for such applications. Indentation size effect (ISE) for TiAl alloys is thoroughly examined using the instrumented nanoindentation technique. It is noted that ISE of TiAl alloys is significantly influenced by the composition, microstructure, and prior deformation characteristics of the system. Prominent ISE is noted for the 3rd generation alloys, as compared to the 2nd generation ones. Theoretical estimations show hardness as a function of geometrically necessary dislocations (GNDs) and statistically stored dislocations. The distribution of GNDs beneath the indentation volume is also experimentally validated. The pre-strained alloys show a lesser extent of ISE and higher bulk hardness owing to the occurrence of preexisting dislocations and twins. The initiation of plasticity at the nanoscale is also dictated by the inherent dislocation characteristics of the alloys. Lower pop-in loads are noticed for pre-strained alloys that are related to the heterogeneous nucleation of dislocations. Overall, this comprehensive study on ISE establishes a correlation between microscopic and bulk hardness and further unravels the distinctive deformation mechanism, active at different length scales from nano to bulk region.
Graphical abstract












Similar content being viewed by others
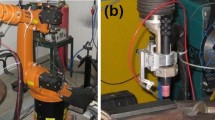
References
Nix WD, Gao H (1998) Indentation size effects in crystalline materials: a law for strain gradient plasticity. J Mech Phys Solids 46:411–425
Motz C, Weygand D, Senger J, Gumbsch P (2008) Micro-bending tests: a comparison between three-dimensional discrete dislocation dynamics simulations and experiments. Acta Mater 56:1942–1955
Palomares-García AJ, Pérez-Prado MT, Molina-Aldareguia JM (2017) Effect of lamellar orientation on the strength and operating deformation mechanisms of fully lamellar TiAl alloys determined by micropillar compression. Acta Mater 123:102–114
Street T, Street O (1994) Strain gradient plasticity: theory and experiment. Acta Metall Mater 42:475–487
Abu Al-Rub RK, Voyiadjis GZ (2004) Analytical and experimental determination of the material intrinsic length scale of strain gradient plasticity theory from micro- and nano-indentation experiments. Int J Plast 20:1139–1182
Elmustafa AA, Stone DS (2003) Nanoindentation and the indentation size effect: kinetics of deformation and strain gradient plasticity. J Mech Phys Solids 51:357–381
Durst K, Backes B, Franke O, Göken M (2006) Indentation size effect in metallic materials: modeling strength from pop-in to macroscopic hardness using geometrically necessary dislocations. Acta Mater 54:2547–2555. https://doi.org/10.1016/j.actamat.2006.01.036
Pharr GM, Herbert EG, Gao Y (2010) The indentation size effect: a critical examination of experimental observations and mechanistic interpretations. Annu Rev Mater Res 40:271–292. https://doi.org/10.1146/annurev-matsci-070909-104456
Durst K, Franke O, Böhner A, Göken M (2007) Indentation size effect in Ni-Fe solid solutions. Acta Mater 55:6825–6833. https://doi.org/10.1016/j.actamat.2007.08.044
Mukhopadhyay NK, Paufler P (2006) Micro- and nanoindentation techniques for mechanical characterisation of materials. Int Mater Rev 51:209–245
Durst K, Backes B, Göken M (2005) Indentation size effect in metallic materials: correcting for the size of the plastic zone. Scr Mater 52:1093–1097. https://doi.org/10.1016/j.scriptamat.2005.02.009
Ma X, Higgins W, Liang Z et al (2021) Exploring the origins of the indentation size effect at submicron scales. Proc Natl Acad Sci USA 118:1–7. https://doi.org/10.1073/pnas.2025657118
Meng Y, Ju X, Yang X (2021) The measurement of the dislocation density using TEM. Mater Charact 175:111065
Graça S, Colaço R, Carvalho PA, Vilar R (2008) Determination of dislocation density from hardness measurements in metals. Mater Lett 62:3812–3814. https://doi.org/10.1016/j.matlet.2008.04.072
Feng G, Nix WD (2004) Indentation size effect in MgO. Scr Mater 51:599–603. https://doi.org/10.1016/j.scriptamat.2004.05.034
Javaid F, Xu Y, Bruder E, Durst K (2018) Indentation size effect in tungsten: quantification of geometrically necessary dislocations underneath the indentations using HR-EBSD. Mater Charact 142:39–42. https://doi.org/10.1016/j.matchar.2018.05.016
Sen I, Roy S, Wagner MF (2017) Indentation response and structure-property correlation in a bimodal Ti–6Al–4V alloy. Adv Eng Mater 19:1700298
Nath P, Marandi L, Sen I (2022) Processing-microstructure-property correlation in thermo-mechanically processed Ti-6Al-4V alloys: a comparative study between conventional and novel approaches. J Alloys Compd 927:167039
Bond T, Badmos A, Ahmed RA et al (2022) Indentation size effects in aluminum and titanium alloys. Mater Sci Eng A 839:142542
Prasitthipayong A, Vachhani SJ, Tumey SJ et al (2018) Indentation size effect in unirradiated and ion-irradiated 800H steel at high temperatures. Acta Mater 144:896–904. https://doi.org/10.1016/j.actamat.2017.11.001
Milman YV, Golubenko AA, Dub SN (2011) Indentation size effect in nanohardness. Acta Mater 59:7480–7487. https://doi.org/10.1016/j.actamat.2011.08.027
Nath P, Scholz F, Pfetzing J et al (2023) Influence of microstructural homogenization on the localized deformation behavior of single-crystal Ni-based superalloy, CMSX-4. Metall Mater Trans A 54:4498–4514. https://doi.org/10.1007/s11661-023-07183-w
Ma X, Li F, Sun Z et al (2019) Strain rate dependence of the indentation size effect in Ti–10V–2Fe–3Al alloy. Mater Sci Technol 35:1107–1113. https://doi.org/10.1080/02670836.2019.1612596
Cui L, Jiang S, Xu J et al (2021) Revealing relationships between microstructure and hardening nature of additively manufactured 316L stainless steel. Mater Des 198:109385
Xu R, Li M, Zhao Y (2022) A Review of microstructure control and mechanical performance optimization of γ-TiAl alloys. J Alloys Compd 932:167611
Maier V, Schunk C, Göken M, Durst K (2015) Microstructure-dependent deformation behaviour of bcc-metals–indentation size effect and strain rate sensitivity. Philos Mag 95:1766–1779
Appel F, Clemens H, Fischer FD (2016) Modeling concepts for intermetallic titanium aluminides. Prog Mater Sci 81:55–124. https://doi.org/10.1016/j.pmatsci.2016.01.001
Bibhanshu N, Rajanna R, Bhattacharjee A, Suwas S (2021) Phase transformations in third generation gamma titanium aluminides: Ti-45Al-(5, 10) Nb-0.2 B-0.2 C. Metall Mater Trans A 52:5300–5313
Dai C, Sun J (2022) Microstructure optimization and improved tensile property in a high Nb-containing γ-TiAl alloy. Mater Charact 185:111743. https://doi.org/10.1016/j.matchar.2022.111743
Appel F, Paul JDH, Oehring M et al (2003) Creep behavior of TiAl alloys with enhanced high-temperature capability. Metall Mater Trans A 34:2149–2164
Niu HZ, Chen YY, Xiao SL et al (2011) High temperature deformation behaviors of Ti-45Al-2Nb-1.5 V-1Mo-Y alloy. Intermetallics 19:1767–1774
Zhu H, Seo DY, Maruyama K, Au P (2006) Strengthening of a fully lamellar TiAl+ W alloy by dynamic precipitation of β phase during long-term creep. Scr Mater 54:425–430
Nath P, Bhattacharjee A, Sen I (2023) Designing of novel microstructure and its impact on the improved service temperature mechanical performance of 2nd and 3rd generation advanced intermetallic TiAl alloys. Mater Sci Eng A 893:146108
Liu Y, Ngan AHW (2001) Depth dependence of hardness in copper single crystals measured by nanoindentation. Scr Mater 44:237–241
Pharr GM, Oliver WC (1992) An improved technique for determining hardness and elastic modulus using load and displacement sensing indentation experiments. J Mater Res 7:1564–1583
Wang Z, Wang S, Zhou S et al (2020) Micro-and macroscopic plastic flow responses in high Nb-containing TiAl alloy by nanoindentation. Intermetallics 127:106958: 1-9
Cha L, Clemens H, Dehm G (2011) Microstructure evolution and mechanical properties of an intermetallic Ti-43.5Al-4Nb-1Mo-0.1B alloy after ageing below the eutectoid temperature. Int J Mater Res 102:703–708. https://doi.org/10.3139/146.110526
Niu HZ, Chen YY, Xiao SL, Xu LJ (2012) Microstructure evolution and mechanical properties of a novel beta γ-TiAl alloy. Intermetallics 31:225–231
Xu X, Ding H, Huang H et al (2021) Role of growth rate on microstructure evolution, element distribution and nanohardness of phases in directionally solidified multiphase high-Nb TiAl alloy. J Mater Res Technol 14:2884–2896. https://doi.org/10.1016/j.jmrt.2021.08.104
Sun F-S, Cao C-X, Yan M-G, Kim S-E (2001) Alloying mechanism of beta stabilizers in a TiAl alloy. Metall Mater Trans A 32:1573–1589
Totten GE, Xie L, Funatani K (2003) Handbook of mechanical alloy design. CRC Press
Yang B, Vehoff H (2007) Dependence of nanohardness upon indentation size and grain size–a local examination of the interaction between dislocations and grain boundaries. Acta Mater 55:849–856
Qiu X, Huang Y, Nix WD et al (2001) Effect of intrinsic lattice resistance in strain gradient plasticity. Acta Mater 49:3949–3958
De Guzman MS, Neubauer G, Flinn P, Nix WD (1993) The role of indentation depth on the measured hardness of materials. In: MRS online proceedings library (OPL)
Ameri AAH, Elewa NN, Ashraf M, Escobedo-Diaz JP (2017) General methodology to estimate the dislocation density from microhardness measurements. Mater Charact 131:324–330
Taylor GI (1938) Plastic strain in metals. J Inst Met 62:307–324
Mattucci MA, Cherubin I, Changizian P et al (2021) Indentation size effect, geometrically necessary dislocations and pile-up effects in hardness testing of irradiated nickel. Acta Mater 207:116702. https://doi.org/10.1016/j.actamat.2021.116702
Taylor GI (1938) Timoshenko Anniversary volume. Macmillan, New York
Dimiduk DM (1999) Gamma titanium aluminide alloys—an assessment within the competition of aerospace structural materials. Mater Sci Eng A 263:281–288
Mecking H, Kocks UF, Hartig C (1996) Taylor factors in materials with many deformation modes. Scr Mater 35:465–471. https://doi.org/10.1016/1359-6462(96)00137-6
Parthasarathy TA, Mendiratta MG, Dimiduk DM (1998) Flow behavior of PST and fully lamellar polycrystals of Ti–48Al in the microstrain regime. Acta Mater 46:4005–4016
Appel F, Paul JDH, Oehring M (2011) Gamma titanium aluminide alloys: science and technology. John Wiley & Sons
Durst K, Neumeier S, Parsa AB et al (2015) Nanoindentation studies of the mechanical properties of the μ phase in a creep deformed Re containing nickel-based superalloy. Mater Sci Eng A 634:202–208. https://doi.org/10.1016/j.msea.2015.03.045
Franke O, Durst K, Göken M (2007) Microstructure and local mechanical properties of Pt-modified nickel aluminides on nickel-base superalloys after thermo-mechanical fatigue. Mater Sci Eng A 467:15–23. https://doi.org/10.1016/j.msea.2007.02.069
Song XP, Chen GL (2001) Determination of the stacking fault energy in high-Nb γ-TiAl. J Mater Sci Lett 20:659–661
Dumitraschkewitz P, Clemens H, Mayer S, Holec D (2017) Impact of alloying on stacking fault energies in γ-TiAl. Appl Sci 7:1193
Calcagnotto M, Ponge D, Demir E, Raabe D (2010) Orientation gradients and geometrically necessary dislocations in ultrafine grained dual-phase steels studied by 2D and 3D EBSD. Mater Sci Eng A 527:2738–2746
Nath P, Kumar SS, Mathews NG et al (2023) Effect of microstructure and temperature on the bulk and small-scale deformation behavior of 2nd and 3rd generation advanced intermetallic TiAl alloys. Mater Charact 207:113585. https://doi.org/10.1016/j.matchar.2023.113585
Kim JY, Kang SK, Greer JR, Kwon D (2008) Evaluating plastic flow properties by characterizing indentation size effect using a sharp indenter. Acta Mater 56:3338–3343. https://doi.org/10.1016/j.actamat.2008.02.049
Shim S, Bei H, George EP, Pharr GM (2008) A different type of indentation size effect. Scr Mater 59:1095–1098
Chen GL, Zhang LC (2002) Deformation mechanism at large strains in a high-Nb-containing TiAl at room temperature. Mater Sci Eng A 329:163–170
Liu S, Ding H, Zhang H et al (2018) High-density deformation nanotwin induced significant improvement in the plasticity of polycrystalline γ-TiAl-based intermetallic alloys. Nanoscale 10:11365–11374
Lodes MA, Hartmaier A, Göken M, Durst K (2011) Influence of dislocation density on the pop-in behavior and indentation size effect in CaF2 single crystals: experiments and molecular dynamics simulations. Acta Mater 59:4264–4273
Kumar S, Kumar IA, Marandi L, Sen I (2020) Assessment of small-scale deformation characteristics and stress-strain behavior of NiTi based shape memory alloy using nanoindentation. Acta Mater 201:303–315
Sen I, Kumar SS (2021) Characterizing stress-strain behavior of materials through nanoindentation. Elasticity of materials. IntechOpen
Pöhl F (2019) Pop-in behavior and elastic-to-plastic transition of polycrystalline pure iron during sharp nanoindentation. Sci Rep 9:1–12
Lu X, Ma Y, Peng D et al (2023) In situ nanomechanical characterization of hydrogen effects on nickel-based alloy 725 under different metallurgical conditions. J Mater Sci Technol 135:156–169
Montagne A, Audurier V, Tromas C (2013) Influence of pre-existing dislocations on the pop-in phenomenon during nanoindentation in MgO. Acta Mater 61:4778–4786
Mason JK, Lund AC, Schuh CA (2006) Determining the activation energy and volume for the onset of plasticity during nanoindentation. Phys Rev B 73:54102
Acknowledgements
Authors gratefully acknowledge DMRL, Hyderabad, for supplying the TiAl pancakes. Authors sincerely thank the Central Research Facility of IIT Kharagpur for providing the required research facilities. Funding for the project by DRDO via ERIP/ER/DG- NSM/990916704/M/01/1743 is gratefully acknowledged.
Author information
Authors and Affiliations
Contributions
PN contributed to conceptualization, methodology, data curation, formal analysis, investigation, writing—original draft, and writing—review and editing. AB contributed to funding acquisition, project administration, resources, supervision, and writing—review and editing. IS contributed to funding acquisition, project administration, resources, supervision, and writing—review and editing.
Corresponding author
Ethics declarations
Conflict of interest
The authors declare that they have no known competing financial interests or personal relationships that could have appeared to influence the work reported in this paper.
Ethical approval
Not applicable.
Additional information
Handling Editor: Naiqin Zhao.
Publisher's Note
Springer Nature remains neutral with regard to jurisdictional claims in published maps and institutional affiliations.
Rights and permissions
Springer Nature or its licensor (e.g. a society or other partner) holds exclusive rights to this article under a publishing agreement with the author(s) or other rightsholder(s); author self-archiving of the accepted manuscript version of this article is solely governed by the terms of such publishing agreement and applicable law.
About this article
Cite this article
Nath, P., Bhattacharjee, A. & Sen, I. Indentation size effect in 2nd and 3rd generation advanced intermetallic TiAl alloys: theoretical and experimental estimation of dislocation density. J Mater Sci 59, 3066–3086 (2024). https://doi.org/10.1007/s10853-023-09320-7
Received:
Accepted:
Published:
Issue Date:
DOI: https://doi.org/10.1007/s10853-023-09320-7