Abstract
Ultra-high speed grinding (UHSG) is a promising machining technology because it can reduce grinding force and greatly increase machining efficiency. However, a mass heat produced in the workpiece-tool contact (WTC) zone risks burning the machined surface during UHSG. Therefore, it is important to ensure timely cooling. The paper studies the flow field of the coolant and cooling capacity of WTC zone under different grinding speeds and coolant velocities by both three-dimensional (3D) finite element (FE) simulation and experiments. Results show that coolant velocity rapidly decreases after a slight initial increase with increasing the distance from the nozzle and little coolant could enter the WTC zone to cool down, which is responsible for the temperature rise in the WTC zone in UHSG. As grinding speed increases, a higher coolant velocity is needed for breaking the barrier of airflow layer to cooling. The critical coolant velocity to break the barrier and enter the WTC zone is obtained by theoretical calculation and experiment. The critical coolant velocity increases with the grinding speed, the coolant velocity needed is 19.2 m/s at the grinding speed of 120 m/s, and it rises to 30 m/s at the grinding speed of 160 m/s. This study could provide a guide to improve the cooling performance in UHSG, which is of great significance to a high-quality surface finish by UHSG.














Similar content being viewed by others
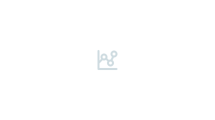
References
Thellaputta GR, Chandra PS, Rao CSP (2017) Machinability of nickel based superalloys: a review. Mater Today Proc 4(2):3712–3721
Ezugwu EO (2005) Key improvements in the machining of difficult-to-cut aerospace superalloys. Int J Mach Tools Manuf 45:1353–1367
Soo SL, Hood R, Aspinwall DK, Voice WE, Sage C (2011) Machinability and surface integrity of RR1000 nickel based superalloy. CIRP Ann Manuf Technol 60(1):89–92
Qiu B, Zhu YJ, Ding WF (2020) An investigation on material removal mechanism in ultra-high-speed grinding of nickel-based superalloy: three-dimensional simulation and experimental verification. Int J Adv Manuf Technol 110:919–933
Thakur A, Gangopadhyay S (2016) State-of-the-art in surface integrity in machining of nickel-based superalloys. Int J Mach Tools Manuf 100:25–54
Li BK, Dai CW, Ding WF, Yang CY, Li CH, Kulik O, Shumyacher V (2020) Prediction on grinding force during grinding powder metallurgy nickel-based superalloy FGH96 with electroplated CBN abrasive wheel. Chin J Aeronaut, Published online. https://doi.org/10.1016/j.cja.2020.05.002
Yeo SH, Ramesh K, Zhong ZW (2002) Ultra-high-speed grinding spindle characteristics upon using oil/air mist lubrication. Int J Mach Tools Manuf 42(7):815–823
Miller S (1996) Advanced materials means advanced engines. Interdiscip Sci Rev 21:117–129
Miao Q, Ding WF, Kuang WJ, Yang CY (2019) Grinding force and surface quality in creep feed profile grinding of turbine blade root of nickel-based superalloy with microcrystalline alumina abrasive wheels. Chin J Aeronaut, Published online. https://doi.org/10.1016/j.cja.2019.11.006
Ezugwu EO, Bonney J, Yamane Y (2003) An overview of the machinability of aeroengine alloys. J Mater Process Technol 134:233–253
Baumgart C, Radziwill JJ, Kuster F, Wegener K (2017) A study of the interaction between coolant jet nozzle flow and the airflow around a grinding wheel in cylindrical grinding. Procedia CIRP 58:517–522
Li CH, Zhang XW, Zhang Q, Wang S, Zhang DK, Jia DZ, Zhang YB (2014) Modeling and simulation of useful fluid flow rate in grinding. Int J Adv Manuf Technol 75(9–12):1587–1604
Engineer F, Guo C, Malkin S (1992) Experimental measurement of fluid flow through the grinding zone. J Eng Ind Trans ASME 114(1):61–66
Hryniewicz P, Szeri AZ, Jahanmir S (2001) Application of lubrication theory to fluid flow in grinding: part I - flow between smooth surfaces. J Tribol - T ASME 123(1):94–100
Tönshoff HK, Friemuth T, Becker JC (2002) Process monitoring in grinding. CIRP Ann Manuf Technol 51(2):551–571
Kharka V, Jain NK, Gupta K (2020) Influence of MQL and hobbing parameters on microgeometry deviations and flank roughness of spur gears manufactured by MQL assisted hobbing. J Mater Res Technol 9(5):9646–9656
Javaroni RL, Lopes JC, Diniz AE, Garcia MV, Ribeiro FSF, Tavares AB, Talon AG, Sanchez LEA, Mello HJ, Aguiar PR, Bianchi EC (2020) Improvement in the grinding process using the MQL technique with cooled wheel cleaning jet. Tribol Int 152:106512
Gaurav G, Sharma A, Dangayach GS, Meena ML (2020) Assessment of jojoba as a pure and nano-fluid base oil in minimum quantity lubrication (MQL) hard-turning of Ti–6Al–4V: a step towards sustainable machining. J Clean Prod 272:122553
Saha S, Deb S, Bandyopadhyay PP (2020) An analytical approach to assess the variation of lubricant supply to the cutting tool during MQL assisted high speed micromilling. J Mater Process Technol 285:116783
Iskandar Y, Tendolkar A, Attia MH, Hendrick P, Damir A, Diakodimitris C (2014) Flow visualization and characterization for optimized MQL machining of composites. CIRP Ann Manuf Technol 63(1):77–80
Leppert T (2011) Effect of cooling and lubrication condition on surface topography and turning process of C45 steel. Int J Mach Tools Manuf 51(2):120–126
Pereira O, Català P, Rodríguez A, Ostra T, Vivancos J, Rivero A, López de Lacalle LN (2015) The use of hybrid CO2+MQL in machining operations. Procedia Eng 132:492–499
Pereira O, Rodríguez A, Ayesta I, García JB, Fernández-Abia AI, López de Lacalle LN (2016) A cryo lubri-coolant approach for finish milling of aeronautical hard-to-cut materials. Int J Pr Eng Man-GT 9(4):370
González H, Calleja A, Pereira O, Ortega N, López de Lacalle LN, Barton M (2018) Super abrasive machining of integral rotary components using grinding flank tools. Metals 8(1):24
Gong L, Bertolini R, Ghiotti A, He N, Bruschi S (2020) Sustainable turning of Inconel 718 nickel alloy using MQL strategy based on graphene nanofluids. Int J Adv Manuf Technol 108:3159–3174
Qian N, Fu YC, Zhang YW, Chen JJ, Xu JH (2019) Experimental investigation of thermal performance of the oscillating heat pipe for the grinding wheel. Int J Heat Mass Transf 136:911–923
He QS, Fu YC, Chen JJ, Zhang W, Cui ZM (2018) Experimental investigation of cooling characteristics in wet grinding using heat pipe grinding wheel. Int J Adv Manuf Technol 97:1–4
Ghorabaee H, Emami MRS, Shafahi M (2019) Effect of nanofluid and surfactant on thermosyphon heat pipe performance. Heat Transfer Eng 41(21):1829–1842
Chen JJ, Fu YC, Gu ZB, Shen HF, He QS (2017) Study on heat transfer of a rotating heat pipe cooling system in dry abrasive-milling. Appl Therm Eng 115:736–743
Ebbrell S, Woolley NH, Tridimas YD, Allanson DR, Rowe WB (2000) The effects of cutting fluid application methods on the grinding process. Int J Mach Tools Manuf 40(2):209–223
Morgan MN, Jackson AR, Wu H, Baines-Jones V, Batako A, Rowe WB (2008) Optimization of fluid application in grinding. CIRP Ann Manuf Technol 57:363–366
Gviniashvili V, Webster J, Rowe B (2005) Fluid flow and pressure in the grinding wheel-workpiece interface. J Manuf Sci E T ASME 127(1):198–205
Hryniewicz P, Szeri AZ, Jahanmir S (2001) Application of lubrication theory to fluid flow in grinding: part II - influence of wheel and workpiece roughness. J Tribol - T ASME 23(1):101–107
Wang CY, Zhang L, Yang CF (2014) Analysis and simulation of air flow field surrounding grinding wheel. Adv Mater Res 1027:12–15
Nadolny K (2015) Small-dimensional sandwich grinding wheels with a centrifugal grinding fluid provision system for traverse internal cylindrical grinding of steel 100Cr6. J Clean Prod 93:354–363
Tawakoli T, Hadad MJ, Sadeghi MH, Daneshi A, Stöckert S, Rasifard A (2009) An experimental investigation of the effects of workpiece and grinding parameters on minimum quantity lubrication - MQL grinding. Int J Mach Tools Manuf 49(12):924–932
Chen H, Zhao J, Dai YX, Wang ZX, Yu TB (2020) Simulation of 3D grinding temperature field by using an improved finite difference method. Int J Adv Manuf Technol 108:11–12
Heinzel C, Kirsch B, Meyer D, Webster J (2020) Interactions of grinding tool and supplied fluid. CIRP Ann Manuf Technol, Published online 69:624–645. https://doi.org/10.1016/j.cirp.2020.05.001
Talon AG, Lopes JC, Sato BK, Tavares AB, Ribeiro FSF, Genovez MC, Pinto TAD, Mello HJ, Aguiar PR, Bianchi EC (2020) Grinding performance of hardened steel: a study about the application of different cutting fluids with corrosion inhibitor. Int J Adv Manuf Technol 108:9–10
Lan SL, Jiao F (2019) Modeling of heat source in grinding zone and numerical simulation for grinding temperature field. Int J Adv Manuf Technol 103:5–8
Komanduri R, Chandrasekaran N, Raff LM (1999) Some aspects of machining with negative-rake tools simulating grinding: a molecular dynamics simulation approach. Philos Mag B 79(7):955–968
Rowe WB (2001) Thermal analysis of high efficiency deep grinding. Int J Mach Tools Manuf 41(1):1–19
Inasaki I, Tönshoff HK, Howes TD (1993) Abrasive machining in the future. CIRP Ann Manuf Technol 42(2):46–49
Engineer GC, Malkin S (1992) Experimental measurement of fluid flow through the grinding zone. J Eng Ind 114(1):61–66
Ramesh K, Huang H, Yin L (2004) Analytical and experimental investigation of coolant velocity in high speed grinding. Int J Mach Tools Manuf 44:1069–1076
Mao C, Zou H, Huang X, Zhang J, Zhou ZX (2013) The influence of spraying parameters on grinding performance for nanofluid minimum quantity lubrication. Int J Adv Manuf Technol 64:1791–1799
Li BK, Miao Q, Li M, Zhang X, Ding WF (2020) An investigation on machined surface quality and tool wear during creep feed grinding of powder metallurgy nickel-based superalloy FGH96 with alumina abrasive wheels. Adv Manuf 8(2):160–176
Xu HJ, Fu YC, Sun FH, Xu XP (2002) Fundamental studies on enhancing heat transfer in contact zone during high efficiency grinding. Sci China Ser E Technol Sci 45(3):261–272
Sato Y, Niceno B (2018) Pool boiling simulation using an interface tracking method: from nucleate boiling to film boiling regime through critical heat flux. Int J Heat Mass Transf 125:876–890
Gorenflo D, Baumhögger E, Windmann T, Herres G (2010) Nucleate pool boiling, film boiling and single-phase free convection at pressures up to the critical state. Part I: integral heat transfer for horizontal copper cylinders. Int J Refrig 33(7):1229–1250
Wang QY, Shi Y, Chen RK (2020) Transition between thin film boiling and evaporation on nanoporous membranes near the kinetic limit. Int J Heat Mass Transf 1544:119673
Funding
This work was financially supported by the National Natural Science Foundation of China (Nos. 51921003 and 51775275), National Key Laboratory of Science and Technology on Helicopter Transmission (Nanjing University of Aeronautics and Astronautics) (No. HTL-A-20G01) and the Project Funded by China Postdoctoral Science Foundation (No. 2020TQ0149).
Author information
Authors and Affiliations
Corresponding author
Additional information
Publisher’s note
Springer Nature remains neutral with regard to jurisdictional claims in published maps and institutional affiliations.
Rights and permissions
About this article
Cite this article
Qiu, B., Yin, J., Ding, W. et al. Flow field and cooling capacity in workpiece-tool contact zone during ultra-high speed grinding. Int J Adv Manuf Technol 111, 2349–2359 (2020). https://doi.org/10.1007/s00170-020-06295-6
Received:
Accepted:
Published:
Issue Date:
DOI: https://doi.org/10.1007/s00170-020-06295-6