Abstract
Background
RNA polymerase II plays critical roles in transcription in eukaryotic organisms. C-terminal Domain Phosphatase-like 1 (CPL1) regulates the phosphorylation state of the C-terminal domain of RNA polymerase II subunit B1, which is critical in determining RNA polymerase II activity. CPL1 plays an important role in miRNA biogenesis, plant growth and stress responses. Although cpl1 mutant showes delayed-flowering phenotype, the molecular mechanism behind CPL1’s role in floral transition is still unknown.
Results
To study the role of CPL1 during the floral transition, we first tested phenotypes of cpl1-3 mutant, which harbors a point-mutation. The cpl1-3 mutant contains a G-to-A transition in the second exon, which results in an amino acid substitution from Glu to Lys (E116K). Further analyses found that the mutated amino acid (Glu) was conserved in these species. As a result, we found that the cpl1-3 mutant experienced delayed flowering under both long- and short-day conditions, and CPL1 is involved in the vernalization pathway. Transcriptome analysis identified 109 genes differentially expressed in the cpl1 mutant, with 2 being involved in floral transition. Differential expression of the two flowering-related DEGs was further validated by qRT-PCR.
Conclusions
Flowering genetic pathways analysis coupled with transciptomic analysis provides potential genes related to floral transition in the cpl1-3 mutant, and a framework for future studies of the molecular mechanisms behind CPL1’s role in floral transition.
Similar content being viewed by others
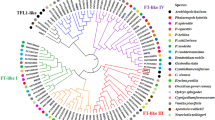
Background
RNA polymerase II (Pol II) is a multiunit enzyme complex that plays critical roles in transcription in eukaryotic organisms. The C-terminal domain (CTD) of its largest subunit, RNA polymerase II subunit B1, recruits regulatory factors required to regulate transcription and RNA processing to RNA Pol II [1]. The Mediator complex integrates general transcription factors and gene-specific activators or repressors to this enzyme complex [2]. The CTD of RNA polymerase II subunit B1 consists of conserved heptad peptide repeats and their phosphorylation states are critical in determining RNA Pol II’s activity level [1, 3]. Many phosphatases play roles in regulating phosphorylation states of RNA polymerase II subunit B1 in yeast, plants and animals [1, 4,5,6]. In Arabidopsis, there are several CTD phosphatases [7,8,9]. Among them, CPL1 (C-terminal Domain Phosphatase-like 1) has been extensively studied in stress-response and gene-expression regulation [7, 9,10,11,12,13], and it specifically dephosphorylates the Ser5 residues of RNA Pol II CTD [14].
In Arabidopsis, the CTD phosphatase CPL1 plays an important role in modulating co-transcriptional pre-mRNA processing, thereby affecting growth and stress responses [15]. CPL1 is involved in responses to salt stress, iron deficiency, abscisic acid treatments and wounding [7, 9, 16, 17]. The mutants of CPL1 have enhanced resistance to a leaf fungal pathogen (Alternaria brassicicola) and an aphid pest (Myzus persicae), which indicates that CPL1 also plays roles in pathogen and pest resistance [18].
CPL1 is essential for miRNA biogenesis [13, 19, 20]. The accuracy of processing primary miRNAs into mature miRNAs in plants is enhanced by SERRATE and HYPONASTIC LEAVES 1 (HYL1), and CPL1 interacts with both proteins [11]. Two serine residues of HYL1 are especially important for HYL1 functions, and hyperphosphorylated HYL1 is inactive [13]. The phosphorylation state of HYL1, and thus its activity level, is regulated by CPL1 [13]. CPL1-mediated HYL1 phosphorylation is regulated by Regulator of CBF Gene Expression 3 (RCF3) [19, 21, 22]. RCF3 interacts with CPL1 in the nucleus, and these interactions are essential to regulate the phosphorylation state of HYL1 [19]. The inactivation of RCF3 causes a phosphorylation shift of HYL1 towards the less active version [22].
Floral transition is one of the most important phase changes in flowering plants, which is regulated by both genetic and environmental factors. There are at least five flowering regulation pathways in Arabidopsis, including photoperiod pathway, vernalization pathway, autonomous pathway, gibberellin pathway and temperature pathway [23,24,25,26,27]. MAF5 is a MADS-box transcription factor that represses floral transition [28], MAF5 is the closest homolog of FLOWERING LOCUS C (FLC), an important repressor in the floral transition pathway [28,29,30]. There are five FLC homologs in Arabidopsis, MAF1–5, which, together with FLC, form a small family of closely related MADS-box transcription factors [31,32,33]. MAF5 is normally repressed and its overexpression causes late-flowering [31]. MAF5 is also involved in the prevention of precocious vernalization responses [34].
Many flowering-time regulators in the vernalization and autonomous pathways promote or inhibit flowering by directly regulating FLC and MAF expression levels [28, 35,36,37], including FRIGIDA (FRI). FRI is a major locus that determines the natural variation in Arabidopsis flowering time [38, 39], and it is responsible for the accelerated transition to flowering after vernalization in Arabidopsis. The plant-specific FRI possesses a coiled-coil domain and forms a large protein complex [38, 40]. FRI, FRIGIDA LIKE 1, FLC EXPRESSOR, FRIGIDA ESSENTIAL 1 and SUPPRESSOR OF FRIGIDA 4 form a complex known as FRIc that acts to promote FLC expression [40,41,42,43].The cpl1 mutants undergo delayed flowering [18], but the molecular mechanism underlying CPL1’s involvement in floral transition is still largely unknown. Here, we found that a mutant harboring a point-mutation, cpl1-3, showed a delayed-flowering phenotype under both long-day and short-day conditions, and genetic pathway analyses revealed that CPL1 was involved in the vernalization pathway. To determine the molecular mechanism behind CPL1’s role in floral transition, a transcriptome analysis was performed. In total, 109 differentially expressed genes were found between wild-type and cpl1-3 mutant seedlings at 9 days after germination. Among them, two DEGs were involved in floral transition. These results provide insights into genes potentially related to floral transition in the cpl1-3 mutant and will aid in further studies of the molecular mechanisms underlying CPL1’s role in floral transition.
Methods
Plant materials and growth conditions
The mutants co-9, ft-10, Col:FRISF2 (FRI-Col), fld-3 and fve-4 were all in the Col background [44, 45]. The fpa-7 (SALK_138449), fca-2 (SALK_057540) and cpl1-3 (CS16351) seeds were bought from the Arabidopsis Biological Resource Center (http://www.arabidopsis.org/). All the plants were grown under long-day (16-h/8-h, light/dark) or short-day (8-h/16-h, light/dark) conditions, at 23 °C and a relative humidity of 75 %. The light intensity at the soil surface was 100 µmol m− 2 s− 1.
Plasmid construction and transgenic plant generation
To construct 35 S:CPL1-3FLAG, the CPL1 coding sequence was amplified and then cloned into the binary vector pCAMBIA1300-35 S:3FLAG. The primers used for plasmid construction are listed in Additional file 1.
Transgenic plants were generated through Agrobacterium tumefaciens-mediated transformation using the floral-dipping method. The cpl1-3 mutants used for transformation were approximately 4 weeks old with plenty of inflorescences. Developing floral tissues were dipped into an Agrobacterium solution containing 5 % sucrose and Silwet-77 (500 µL L− 1). Transformants containing 35 S:CPL1-3FLAG were selected on MS medium supplemented with hygromycin (30 mg L− 1). Two independent homozygous T3 lines with hygromycin resistance were chosen for further studies.
Cleaved Amplified Polymorphic Sequences (CAPS) Analysis
A 610-bp DNA fragment of WT or the cpl1-3 mutant was amplified using the following primers: Forward, 5′-TCTGGCGAGAGGTGTCC-3′/Reverse, 5′-GCTGAAACCCGTCAATCTTAT-3′. PCR was carried out as follows: 40 cycles of 95 °C for 30 s, 58 °C for 30 s and 72 °C for 1 min. Then, the PCR products were digested by Sac I and separated on 1 % agarose-TAE gels.
Flowering-time measurement
The flowering times of the plants were measured by assessing the numbers of rosette leaves and the number of days when the first flowers appeared.
Transcriptomic analyses
Total RNA was isolated using an RNAprep Pure Plant Kit (TIANGEN, Beijing, China), and then, DNase I was added to eliminate genomic and plastid DNA. The isolated total RNA was analyzed using a NanoDrop and Agilent 2100 Bioanalyzer (Thermo Fisher Scientific, MA, USA). mRNA was purified using Oligo (dT) magnetic beads and then sheared into small fragments. The first-strand cDNA was reverse transcribed using random hexamer primers, followed by second-strand cDNA synthesis. Then, an A-Tailing Mix and RNA Index Adapters were added. The resultant cDNA fragments were amplified by PCR, and then dissolved in EB solution. The double-stranded PCR products were heat denatured to produce the final library. The sequencing was performed on a BGIseq500 platform (BGI-Shenzhen, China). The transcriptome data were filtered and analyzed in accordance with a previous paper [46]. Differential expression analyses were performed using the following criteria: |log2(-fold change) | > 1 and Q value < 0.05.
qRT-PCR
In total, 1 µg RNA was reverse transcribed using a FastKing gDNA Dispelling RT SuperMix kit (TIANGEN) in accordance with the manufacturer’s instructions. The qRT-PCR was performed using an UltraSYBR Mixture (with ROX; CWBio, Beijing, China) and the CFX96 real-time PCR detection system (Bio-Rad, CA, USA). The expression of TUBULIN 2 (TUB2) was used as an internal control. Error bars denote SD of three biological replicates. All the primers used for qRT-PCR are listed in Additional file 1.
Statistical analysis
The experimental data were analyzed using two-tailed paired Student’s t tests with SPSS 12.0 software.
Results
Loss of CPL1 function delays flowering in Arabidopsis
CPL1 regulates flowering time in Arabidopsis [18], but the molecular mechanism underlying CPL1’s role in floral transition is still unknown. To elucidate this, we used the cpl1-3 mutant harboring a point-mutation to examine the flowering phenotype [17]. The cpl1-3 mutant contains a G-to-A transition in the second exon (Fig. 1a), which results in an amino acid substitution from Glu to Lys (E116K) (Additional file 2) and leads to the loss of a Sac I site in the CPL1 gene (Fig. 1b). We then analyzed CPL protein sequence of Arabidopsis and other different species, and found that the mutated amino acid (Glu) was conserved in these species (Additional file 3). The cpl1-3 mutant displayed a delayed-flowering phenotype under both long- and short-day conditions (Fig. 1d–g), suggesting that CPL1 acts as an activator in floral transition.
CPL1 regulates flowering time in Arabidopsis. a The structure of the CPL1 coding region. Black boxes, gray boxes and black lines represent exons, untranslated regions and introns, respectively. The point mutation is shown below. Red arrowheads indicate the positions of the primers used in Fig. 1b. b A cropped gel of the CAPS analysis of wild-type and the cpl1-3 mutant. Genomic DNA of wild-type (WT) and the cpl1-3 mutant were amplified using CAPS markers listed in Additional file 1, and then, the PCR products were digested with Sac I. c cpl1-3 mutant shows delay-flowering phenotype under long-day conditions. Scale bar: 2 cm. d and e Rosette leaf numbers (d) and days to bolting (e) of the cpl1-3 mutant grown under long-day conditions. f and g Rosette leaf numbers (f) and days to bolting (g) of the cpl1-3 mutant grown under short-day conditions. Values are representative of at least 15 plants showing specific genotypes. Asterisks indicate significant differences between WT and the cpl1-3 mutant in flowering time (Student’s t test, P < 0.05). h cpl1-1-3 35 S:CPL1-3FLAG exhibited a flowering time comparable to that of WT plants under long-day conditions. Scale bar: 2 cm. i and j Rosette leaf numbers (i) and days to bolting (j) of cpl1-1-3 35 S:CPL1-3FLAG grown under long-day conditions. Values are representative of at least 15 plants showing specific genotypes. Asterisks indicate significant differences between WT and the cpl1-3 mutant in flowering time (Student’s t test, P < 0.05)
To confirm the loss of CPL1 function was responsible for the delayed-flowering phenotype of the cpl1-3 mutant, we transformed the cpl1-3 mutant with a construct containing the coding sequence of CPL1 driven by the constitutive cauliflower mosaic virus 35 S promoter. Two independent cpl1-1-3 35 S:CPL1-3FLAG transgenic lines exhibited comparable flowering times to WT plants (Fig. 1 h-j; Additional file 4), indicating that CPL1 was responsible for the flowering phenotype of the cpl1-3 mutant and that excess amounts of CPL1 do not further accelerate flowering.
We further analyzed the CPL1 expression in different tissues of WT plants. The qRT–PCR results showed that CPL1 was mainly expressed in leaves (Additional file 5).
CPL1 is involved in the vernalization pathway
Because CPL1 regulates flowering time in Arabidopsis, we examined whether CPL1 had roles in the flowering-related genetic pathways. The CPL1 expression level did not significantly change in photoperiod pathway mutants (Fig. 2a), FT and CO expression were also not changed in WT and cpl1-3 mutant seedlings (Fig. 2b), and the cpl1-3 mutant flowered late, compared with WT, under both long- and short-day conditions (Fig. 1c–e), indicating that CPL1 may not be involved in the photoperiod pathway. A gibberellin treatment did not alter the CPL1 expression level (Fig. 2c), indicating that CPL1 may not be involved in the gibberellin pathway. The generation of autonomous pathway mutants did not significantly disrupt the CPL1 expression level (Fig. 2d), while FPA, FCA, FLD and FVE expression were all consistent in WT and cpl1-3 mutant seedlings (Fig. 2e), indicating that CPL1 may not be involved in the autonomous pathway. We then used FRI-Col seedlings, which contained FRIGIDA (FRI) in the Col background and elevated FLC transcript level, for further analyses [42]. However, after a vernalization treatment, the CPL1 expression level was elevated in both WT and FRI-Col seedlings (Fig. 2f), suggested that CPL1 may play roles in the vernalization pathway. Furthermore, the CPL1 expression level declined in FRI-Col seedlings compared with in WT seedlings independent of the vernalization treatment (Fig. 2f). This indicated that FRI repressed CPL1 expression. The results suggest that CPL1 may be involved in the vernalization pathway.
CPL1 expression is regulated by the vernalization pathway. a CPL1 expression in photoperiod-pathway mutants at 9 DAG. b FT and CO expression in WT and cpl1-3 mutant seedlings. c CPL1 expression after a gibberellin treatment. The WT seedlings were grown under short-day conditions for 2 weeks and then treated with 100 µM gibberellic acid or 0.1 % ethanol weekly. After 3 weeks (W3) and 5 weeks (W5), samples were collected for further analyses. d CPL1 expression in autonomous-pathway mutants at 9 DAG. e FPA, FCA, FLD and FVE expression in WT and cpl1-3 mutant seedlings. f CPL1 expression after the vernalization treatment. The seeds were vernalized at 4 °C for 8 weeks, and 9-day-old seedlings were collected for further analyses. Asterisks indicate significant differences (Student’s t test, *P < 0.05, **P < 0.01, “ns” indicates statistically not siginificant)
Transcriptome analysis and DEG Identification between WT and the cpl1-3 Mutant
To identify the downstream flowering-time regulators that are responsible for the function of CPL1 in floral transition, RNA-seq analyses were performed. Total RNA isolated from WT and cpl1-3 mutant seedling at 9 DAG were used to construct libraries. Three biological replicates were used, and six libraries were constructed for transcriptome sequencing. The detailed information on the RNA-seq reads used for constructing the six libraries are shown in Additional file 6. Briefly, 271.97 million raw reads were generated. After qualifying and filtering, approximately 267.24 million clean reads (approximately 98.3 %), comprising 40.08 Gb of sequence data, were used for further studies. Over 95.93 % of the clean reads had quality scores at the Q20 level, and over 86.69 % of the clean reads have quality scores at the Q30 level.
As a result, 109 DEGs between WT and the cpl1-3 mutant meeting the criteria |log2(-fold change)| > 1 and Q value < 0.05 (Fig. 3a) were identified and analyzed. Among them, 87 DEGs were up-regulated and 22 DEGs were down-regulated (Fig. 3b). A heatmap of the DEG expression profiles clearly revealed that the samples were separated into two clusters, indicating that the three biological replicates of WT and cpl1-3 mutant were highly repeatable (Fig. 3c). An analysis of the biological functions of these DEGs was performed. For the GO classification, the top five largest GO terms in biological process were “cellular process”, “metabolic process”, “response to stimulus”, “biological regulation”, and “regulation of biological process”; in cellular component, the top five largest GO terms were “cell”, “cell part”, “membrane”, “organelle” and “membrane part”; and in molecular function, “binding”, “catalytic activity” were the two largest GO terms (Fig. 3d).
Transcriptional profiles in WT and cpl1-3 mutant seedlings. a Significance analysis of all the DEGs between WT and cpl1-3 mutant seedlings displayed using a volcano plot. b The numbers of genes that were up- and down-regulated between WT and cpl1-3 mutant seedlings. c Expression profiles of the differentially expressed genes between WT and cpl1-3 mutant seedlings shown using a heatmap. d GO enrichment analysis of DEGs between WT and cpl1-3 mutant seedlings
Identification of Flowering-time-related DEGs and Validation of RNA-seq Data
To investigate the molecular mechanisms underlying CPL1’s role in floral transition, we analyzed the DEGs to determine which were involved in floral transition and responsible for the flowering phenotype of the cpl1-3 mutant. Among the 109 DEGs between WT and the cpl1-3 mutant, 2 DEGs were involved in flowering-related genetic pathways. The expression level of MADS AFFECTING FLOWERING 5 (MAF5) was up-regulated, whereas that of TWIN SISTER OF FT (TSF) was down-regulated in cpl1-3 mutant seedlings (Additional file 7).
To validate the DEG expression levels identified by RNA-seq, qRT-PCR was performed. As shown in Fig. 4a, b, the qRT-PCR results were consistent with RNA abundance levels inferred from the RNA-seq experiments. This result suggests that the RNA-seq data are reliable.
Discussion
CPL1 plays critical roles in transcriptional regulation, and is thus involved in miRNA biogenesis, plant growth and stress responses. In this study, we found that the loss of CPL1 function resulted in a delayed-flowering phenotype in Arabidopsis (Fig. 1). To investigate the molecular mechanisms underlying CPL1’s role in floral transition, transcriptomic analyses between WT and cpl1-3 mutant seedlings were performed. As a result, 109 DEGs were revealed. Among them, two DEGs, MAF5 and TSF, functioned in floral transition (Fig. 4; Additional file 7).
In this study, we found that FRI repressed the expression of CPL1, and a vernalization treatment induced the expression of CPL1 in both WT and FRI-Col seedlings (Fig. 2f). These results indicated that CPL1 plays roles in the vernalization pathway and acts downstream of FRI. This, together with the up-regulated expression of MAF5 in cpl1-3 mutant seedlings (Fig. 4; Additional file 7), led us to speculate that FRI may upregulate MAF5 expression and that responses to vernalization may require functional CPL1.
CPL1 is a phosphatase that can regulate the phosphorylation state of many proteins, including RNA polymerase II subunit B1 and HYL1 [13, 14]. In this study, as the MAF5 expression was up-regulated in cpl1-3 mutant seedlings, indicated that CPL1 may interact with other proteins which regulate the expression of MAF5, and then regulated the phosphorylation state of these proteins and thus their activity levels in regulating MAF5 expression. Further studies should focus on screening CPL1-interacting proteins which may be response for the regulation of MAF5 expression.
In Arabidopsis, TSF is the closest homolog of FLOWERING LOCUS T (FT), sharing an approximately 82 % amino acid sequence identity [47, 48]. TSF and FT expressed in rootstock plants accelerate the flowering of grafted tsf or ft mutant scions, indicating that TSF and FT act through a similar mechanism of protein movement towards the shoot apex, which triggers flowering. The effect of TSF on triggering flowering in mutant scions was weaker than that of FT [49], perhaps because TSF is less mobile than FT. In Arabidopsis, FLC directly suppresses the expression of floral pathway integrators, such as FT [50, 51]. In this study, the MAF5 expression was up-regulated, while TSF expression was down-regulated, in cpl1-3 mutant seedlings, we supposed that MAF5 may also suppress the expression of TSF, but we also cannot exclude the possibility that CPL1 directly regulates the expression of TSF by interacting with other proteins, further studies should focus on this.
Conclusions
In summary, a transcriptome analysis was performed between wild-type and cpl1-3 mutant seedlings at 9 DAG. Through bioimformatics mining, 109 differentially expressed genes were identified, with two genes, MAF5 and TSF, were involved in floral transition. Differential expression of the two flowering-related DEGs was further validated by qRT-PCR. Furthermore, CPL1 expression decreased in FRI seedlings, whereas a vernalization treatment induced CPL1 expression (Fig. 2f). Considering that the expression level of MAF5, the closest homolog of FLC, increased in cpl1-3 seedlings (Fig. 4; Additional file 7), and that the cpl1-3 mutant displayed a delayed-flowering phenotype under both long- and short-day conditions (Fig. 1c–g), we propose that FRI may regulate MAF5 expression through CPL1 and may subsequently suppress downstream TSF to delay flowering. We envisage that further studies on how CPL1 impacts on FRI to regulate MAF5 expression will deepen our knowledge into the vernalization pathway in control of flowering.
Availability of data and materials
The datasets supporting the conclusions of this article are available in the NCBI Short Read Archive with accession number PRJNA699075 (https://www.ncbi.nlm.nih.gov/bioproject/?term=prjna699075).
Abbreviations
- CAPS:
-
Cleaved amplified polymorphic sequences
- CPL1:
-
C-terminal Domain Phosphatase-like 1
- CTD:
-
C-terminal domain
- DAG:
-
Days after germination
- DEGs:
-
Differentially expressed genes
- FLC:
-
FLOWERING LOCUS C
- FRI:
-
FRIGIDA
- FT:
-
FLOWERING LOCUS T
- GA:
-
Gibberellin
- HYL1:
-
HYPONASTIC LEAVES 1
- LD:
-
Long-day
- MAF5:
-
MADS AFFECTING FLOWERING 5
- qRT-PCR:
-
quantitative real-time PCR
- Pol II:
-
RNA polymerase II
- RCF3:
-
Regulator of CBF Gene Expression 3
- RNA-seq:
-
RNA sequencing
- SD:
-
Short-day
- TSF:
-
TWIN SISTER OF FT
- TUB2:
-
TUBULIN 2
- WT:
-
wild-type
References
Hsin JP, Manley JL. The RNA polymerase II CTD coordinates transcription and RNA processing. Genes Dev. 2012;26(19):2119–37.
Zaborowska J, Egloff S, Murphy S. The pol II CTD: new twists in the tail. Nat Struct Mol Biol. 2016;23(9):771–7.
Harlen KM, Trotta KL, Smith EE, Mosaheb MM, Fuchs SM, Churchman LS. Comprehensive RNA polymerase II interactomes reveal distinct and varied roles for each phospho-CTD residue. Cell Rep. 2016;15(10):2147–58.
Egloff S, Dienstbier M, Murphy S. Updating the RNA polymerase CTD code: adding gene-specific layers. Trends Genet. 2012;28(7):333–41.
Eick D, Geyer M. The RNA polymerase II carboxy-terminal domain (CTD) code. Chem Rev. 2013;113(11):8456–90.
Hajheidari M, Koncz C, Eick D: Emerging roles for RNA polymerase II CTD in Arabidopsis. Trends Plant Sci. 2013, 18(11):633–643.
Koiwa H, Barb AW, Xiong L, Li F, McCully MG, Lee BH, Sokolchik I, Zhu J, Gong Z, Reddy M et al: C-terminal domain phosphatase-like family members (AtCPLs) differentially regulate Arabidopsis thaliana abiotic stress signaling, growth, and development. Proc Natl Acad Sci U S A. 2002, 99(16):10893–10898.
Li F, Cheng C, Cui F, de Oliveira MV, Yu X, Meng X, Intorne AC, Babilonia K, Li M, Li B, et al. Modulation of RNA polymerase II phosphorylation downstream of pathogen perception orchestrates plant immunity. Cell Host Microbe. 2014;16(6):748–58.
Xiong L, Lee H, Ishitani M, Tanaka Y, Stevenson B, Koiwa H, Bressan RA, Hasegawa PM, Zhu JK. Repression of stress-responsive genes by FIERY2, a novel transcriptional regulator in Arabidopsis. Proc Natl Acad Sci U S A. 2002;99(16):10899–904.
Chen T, Cui P, Chen H, Ali S, Zhang S, Xiong L. A KH-domain RNA-binding protein interacts with FIERY2/CTD phosphatase-like 1 and splicing factors and is important for pre-mRNA splicing in Arabidopsis. PLoS Genet. 2013;9(10):e1003875.
Jeong IS, Aksoy E, Fukudome A, Akhter S, Hiraguri A, Fukuhara T, Bahk JD, Koiwa H. Arabidopsis C-terminal domain phosphatase-like 1 functions in miRNA accumulation and DNA methylation. PLoS One. 2013;8(9):e74739.
Jiang J, Wang B, Shen Y, Wang H, Feng Q, Shi H. The arabidopsis RNA binding protein with K homology motifs, SHINY1, interacts with the C-terminal domain phosphatase-like 1 (CPL1) to repress stress-inducible gene expression. PLoS Genet. 2013;9(7):e1003625.
Manavella PA, Hagmann J, Ott F, Laubinger S, Franz M, Macek B, Weigel D. Fast-forward genetics identifies plant CPL phosphatases as regulators of miRNA processing factor HYL1. Cell. 2012;151(4):859–70.
Zhang B, Yang G, Chen Y, Zhao Y, Gao P, Liu B, Wang H, Zheng ZL. C-terminal domain (CTD) phosphatase links Rho GTPase signaling to Pol II CTD phosphorylation in Arabidopsis and yeast. Proc Natl Acad Sci U S A. 2016;113(50):E8197–206.
Koiwa H, Hausmann S, Bang WY, Ueda A, Kondo N, Hiraguri A, Fukuhara T, Bahk JD, Yun DJ, Bressan RA, et al. Arabidopsis C-terminal domain phosphatase-like 1 and 2 are essential Ser-5-specific C-terminal domain phosphatases. Proc Natl Acad Sci U S A. 2004;101(40):14539–44.
Aksoy E, Jeong IS, Koiwa H. Loss of function of Arabidopsis C-terminal domain phosphatase-like1 activates iron deficiency responses at the transcriptional level. Plant Physiol. 2013;161(1):330–45.
Matsuda O, Sakamoto H, Nakao Y, Oda K, Iba K. CTD phosphatases in the attenuation of wound-induced transcription of jasmonic acid biosynthetic genes in Arabidopsis. Plant J. 2009;57(1):96–108.
Thatcher LF, Foley R, Casarotto HJ, Gao LL, Kamphuis LG, Melser S, Singh KB. The Arabidopsis RNA Polymerase II Carboxyl Terminal Domain (CTD) Phosphatase-Like1 (CPL1) is a biotic stress susceptibility gene. Sci Rep. 2018;8(1):13454.
Chen T, Cui P, Xiong L. The RNA-binding protein HOS5 and serine/arginine-rich proteins RS40 and RS41 participate in miRNA biogenesis in Arabidopsis. Nucleic Acids Res. 2015;43(17):8283–98.
Cui P, Chen T, Qin T, Ding F, Wang Z, Chen H, Xiong L. The RNA Polymerase II C-Terminal Domain Phosphatase-Like Protein FIERY2/CPL1 Interacts with eIF4AIII and Is Essential for Nonsense-Mediated mRNA Decay in Arabidopsis. Plant Cell. 2016;28(3):770–85.
Jeong IS, Fukudome A, Aksoy E, Bang WY, Kim S, Guan Q, Bahk JD, May KA, Russell WK, Zhu J, et al. Regulation of abiotic stress signalling by Arabidopsis C-terminal domain phosphatase-like 1 requires interaction with a k-homology domain-containing protein. PLoS One. 2013;8(11):e80509.
Karlsson P, Christie MD, Seymour DK, Wang H, Wang X, Hagmann J, Kulcheski F, Manavella PA. KH domain protein RCF3 is a tissue-biased regulator of the plant miRNA biogenesis cofactor HYL1. Proc Natl Acad Sci U S A. 2015;112(45):14096–101.
Amasino RM, Michaels SD. The timing of flowering. Plant Physiol. 2010;154(2):516–20.
Andres F, Coupland G. The genetic basis of flowering responses to seasonal cues. Nat Rev Genet. 2012;13(9):627–39.
Mouradov A, Cremer F, Coupland G. Control of flowering time: interacting pathways as a basis for diversity. Plant Cell. 2002;14(Suppl):S111-130.
Simpson GG, Dean C. Arabidopsis, the Rosetta stone of flowering time? Science. 2002;296(5566):285–9.
Srikanth A, Schmid M. Regulation of flowering time: all roads lead to Rome. Cell Mol Life Sci. 2011;68(12):2013–37.
Mahrez W, Shin J, Munoz-Viana R, Figueiredo DD, Trejo-Arellano MS, Exner V, Siretskiy A, Gruissem W, Kohler C, Hennig L. BRR2a affects flowering time via FLC splicing. PLoS Genet. 2016;12(4):e1005924.
He Y: Control of the transition to flowering by chromatin modifications. Molecular Plant. 2009, 2(4):554–564.
Rataj K, Simpson GG: Message ends: RNA 3’ processing and flowering time control. J Exp Bot. 2014, 65(2):353–363.
Kim DH, Sung S: The Plant Homeo Domain finger protein, VIN3-LIKE 2, is necessary for photoperiod-mediated epigenetic regulation of the floral repressor, MAF5. Proc Natl Acad Sci U S A. 2010, 107(39):17029–17034.
Parenicova L, de Folter S, Kieffer M, Horner DS, Favalli C, Busscher J, Cook HE, Ingram RM, Kater MM, Davies B et al: Molecular and phylogenetic analyses of the complete MADS-box transcription factor family in Arabidopsis: new openings to the MADS world. Plant Cell. 2003, 15(7):1538–1551.
Ratcliffe OJ, Nadzan GC, Reuber TL, Riechmann JL: Regulation of flowering in Arabidopsis by an FLC homologue. Plant Physiol. 2001, 126(1):122–132.
Kim DH, Sung S. Genetic and epigenetic mechanisms underlying vernalization. The Arabidopsis Book. 2014;12:e0171. American Society of Plant Biologists.
He Y: Chromatin regulation of flowering. Trends Plant Sci 2012, 17(9):556–562.
Michaels SD. Flowering time regulation produces much fruit. Curr Opin Plant Biol. 2009;12(1):75–80.
Yu X, Michaels SD. The Arabidopsis Paf1c complex component CDC73 participates in the modification of FLOWERING LOCUS C chromatin. Plant Physiol. 2010;153(3):1074–84.
Johanson U, West J, Lister C, Michaels S, Amasino R, Dean C. Molecular analysis of FRIGIDA, a major determinant of natural variation in Arabidopsis flowering time. Science. 2000;290(5490):344–7.
Michaels SD, He Y, Scortecci KC, Amasino RM. Attenuation of FLOWERING LOCUS C activity as a mechanism for the evolution of summer-annual flowering behavior in Arabidopsis. Proc Natl Acad Sci U S A. 2003;100(17):10102–7.
Choi K, Kim J, Hwang HJ, Kim S, Park C, Kim SY, Lee I. The FRIGIDA complex activates transcription of FLC, a strong flowering repressor in Arabidopsis, by recruiting chromatin modification factors. Plant Cell. 2011;23(1):289–303.
Kim SY, Michaels SD. SUPPRESSOR OF FRI 4 encodes a nuclear-localized protein that is required for delayed flowering in winter-annual Arabidopsis. Development. 2006;133(23):4699–707.
Michaels SD, Bezerra IC, Amasino RM. FRIGIDA-related genes are required for the winter-annual habit in Arabidopsis. Proc Natl Acad Sci U S A. 2004;101(9):3281–5.
Schmitz RJ, Hong L, Michaels S, Amasino RM. FRIGIDA-ESSENTIAL 1 interacts genetically with FRIGIDA and FRIGIDA-LIKE 1 to promote the winter-annual habit of Arabidopsis thaliana. Development. 2005;132(24):5471–8.
Gong X, Shen L, Peng YZ, Gan Y, Yu H. DNA Topoisomerase Ialpha Affects the Floral Transition. Plant Physiol. 2017;173(1):642–54.
Liu L, Li C, Teo ZWN, Zhang B, Yu H. The MCTP-SNARE complex regulates florigen transport in Arabidopsis. Plant Cell. 2019;31(10):2475–90.
Wang J, Xue Z, Lin J, Wang Y, Ying H, Lv Q, Hua C, Wang M, Chen S, Zhou B. Proline improves cardiac remodeling following myocardial infarction and attenuates cardiomyocyte apoptosis via redox regulation. Biochem Pharmacol. 2020;178:114065.
Jang S, Torti S, Coupland G. Genetic and spatial interactions between FT, TSF and SVP during the early stages of floral induction in Arabidopsis. Plant J. 2009;60(4):614–25.
Yamaguchi A, Kobayashi Y, Goto K, Abe M, Araki T. TWIN SISTER OF FT (TSF) acts as a floral pathway integrator redundantly with FT. Plant Cell Physiol. 2005;46(8):1175–89.
Jin S, Jung HS, Chung KS, Lee JH, Ahn JH. FLOWERING LOCUS T has higher protein mobility than TWIN SISTER OF FT. J Exp Bot. 2015;66(20):6109–17.
Hepworth SR, Valverde F, Ravenscroft D, Mouradov A, Coupland G. Antagonistic regulation of flowering-time gene SOC1 by CONSTANS and FLC via separate promoter motifs. EMBO J. 2002;21(16):4327–37.
Searle I, He Y, Turck F, Vincent C, Fornara F, Krober S, Amasino RA, Coupland G. The transcription factor FLC confers a flowering response to vernalization by repressing meristem competence and systemic signaling in Arabidopsis. Genes Dev. 2006;20(7):898–912.
Acknowledgements
We are grateful to BGI (Shenzhen, China) for technical support and Prof. Yiguo Hong for reading this manuscript.
Funding
This work was supported by the National Natural Science Foundation of China (grant no. 31770344; 31970328). The funding body played no role in this study.
Author information
Authors and Affiliations
Contributions
C.Y. and C.Q. designed the experiments. C.Y., J.X., Q.C., Q.L. and Y.H. performed the experiments, C.Y. and Q.L. carried out the qRT-PCR analysis. C.Y., Y.J. and C.Q. analyzed the data. C.Q. drafted the manuscript. All authors read and approved the final manuscript.
Corresponding author
Ethics declarations
Ethics approval and consent to participate
The fpa-7 (SALK_138449), fca-2 (SALK_057540) and cpl1-3 (CS16351) seeds were bought from the Arabidopsis Biological Resource Center (http://www.arabidopsis.org/). The authors declare that they comply with the IUCN Policy Statement on Research Involving Species at Risk of Extinction and the Convention on the Trade in Endangered Species of Wild Fauna and Flora.
Consent for publication
Not applicable.
Competing interests
The authors declare that they have no competing interests.
Additional information
Publisher’s Note
Springer Nature remains neutral with regard to jurisdictional claims in published maps and institutional affiliations.
Supplementary Information
Additional file 1.
The primers used in this study.
Additional file 2.
Alignment of WT and mutant CPL1 protein sequences.
Additional file 3.
Alignment of CPL protein sequence of Arabidopsis and other different species. Red rectangle indicates the position of mutated amino acid (Glu).
Additional file 4.
TheCPL1expression level in independent CPL1-overexpression lines. Seedlings were collected at 9 DAG. The levels of gene expression normalized to TUB2 expression are shown as relative values to that of WT set at 1. Error bars indicate SD of three biological replicates.
Additional file 5.
TheCPL1 expression level in various tissues of WT plants as assessed by qRT-PCR. JRL, juvenile rosette leaves; Rt, roots; ARL, adult rosette leaves; CL, cauline leaves; FL, flowers; St, inflorescence stems; Sil, siliques. Expression levels are shown as relative values to the maximal level set at 100%. Error bars indicate SD of three biological replicates.
Additional file 6.
The detailed information on raw reads from different samples.
Additional file 7.
The list of 109 DEGs between WT and the cpl1-3 mutant.
Additional file 8.
The list of vernalization related genes between WT and the cpl1-3 mutant.
Additional file 9.
The original, full-length gel displayed in Fig. 1b.
Rights and permissions
Open Access This article is licensed under a Creative Commons Attribution 4.0 International License, which permits use, sharing, adaptation, distribution and reproduction in any medium or format, as long as you give appropriate credit to the original author(s) and the source, provide a link to the Creative Commons licence, and indicate if changes were made. The images or other third party material in this article are included in the article's Creative Commons licence, unless indicated otherwise in a credit line to the material. If material is not included in the article's Creative Commons licence and your intended use is not permitted by statutory regulation or exceeds the permitted use, you will need to obtain permission directly from the copyright holder. To view a copy of this licence, visit http://creativecommons.org/licenses/by/4.0/. The Creative Commons Public Domain Dedication waiver (http://creativecommons.org/publicdomain/zero/1.0/) applies to the data made available in this article, unless otherwise stated in a credit line to the data.
About this article
Cite this article
Yuan, C., Xu, J., Chen, Q. et al. C-terminal domain phosphatase-like 1 (CPL1) is involved in floral transition in Arabidopsis. BMC Genomics 22, 642 (2021). https://doi.org/10.1186/s12864-021-07966-8
Received:
Accepted:
Published:
DOI: https://doi.org/10.1186/s12864-021-07966-8