Abstract
Background
Distant hybridization can generate changes in phenotypes and genotypes that lead to the formation of new hybrid lineages with genetic variation. In this study, the establishment of two bisexual fertile carp lineages, including the improved diploid common carp (IDC) lineage and the improved diploid scattered mirror carp (IDMC) lineage, from the interspecific hybridization of common carp (Cyprinus carpio, 2n = 100) (♀) × blunt snout bream (Megalobrama amblycephala, 2n = 48) (♂), provided a good platform to investigate the genetic relationship between the parents and their hybrid progenies.
Result
In this study, we investigated the genetic variation of 12 Hox genes in the two types of improved carp lineages derived from common carp (♀) × blunt snout bream (♂). Hox gene clusters were abundant in the first generation of IDC, but most were not stably inherited in the second generation. In contrast, we did not find obvious mutations in Hox genes in the first generation of IDMC, and almost all the Hox gene clusters were stably inherited from the first generation to the second generation of IDMC. Interestingly, we found obvious recombinant clusters of Hox genes in both improved carp lineages, and partially recombinant clusters of Hox genes were stably inherited from the first generation to the second generation in both types of improved carp lineages. On the other hand, some Hox genes were gradually becoming pseudogenes, and some genes were completely pseudogenised in IDC or IDMC.
Conclusions
Our results provided important evidence that distant hybridization produces rapid genomic DNA changes that may or may not be stably inherited, providing novel insights into the function of hybridization in the establishment of improved lineages used as new fish resources for aquaculture.
Similar content being viewed by others
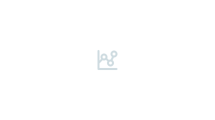
Background
Hybridization may cause interactions involving a wide range of types and levels of genetic divergence between the parental forms [1]. In nature, hybridization among species is reasonably common on a per-species basis, even though it is usually very rare on a per-individual basis. On a per-individual basis, the isolation mechanisms (e.g., reproductive barriers) prevented the occurrence of high frequency hybridization events among individuals of different species. Although hybrids are rare in populations, a few hybrids can provide a bridge to allow a trickle of alleles to pass between species. Thus, if species that hybridize are common, even low rates of hybridization per individual can have important evolutionary consequences in a high fraction of species. It was found that approximately 10–30% of multicellular animal and plant species hybridize regularly [2]. Hybridization among species can act as an additional, perhaps more abundant, source of adaptive genetic variation than mutation (very rare, approximately 10− 8 to 10− 9 per generation per base pair) [3,4,5,6,7]. For example, in Darwin’s finches, ‘New additive genetic variance introduced by hybridization is estimated to be two to three orders of magnitude greater than that introduced by mutation’ [3]. In both plants and animals, distant hybridization appears to facilitate speciation and adaptive radiation [8]. Hybridization has played a key role in recombining the adaptive traits of two species and generating novel phenotypes [9]. For example, common wheat (Triticum aestivum), originated from hybridization between T. turgidum and Aegilops tauschii, has significantly increased grain yield and the harvest index [10]; another plant hybrid is derived from the interspecific hybridization between Vigna umbellata (♀) and V. exilis (♂), which is tolerant to drought and presents early flowering [11]. In Cyprinidae, the autotetraploid hybrids, originated from hybridization between red crucian carp (Carassius auratus red var., ♀) × blunt snout bream (Megalobrama amblycephala, ♂), has significantly shortened the age of sexual maturity compared to their allotetraploid parents [12]; the hybrids derived from blunt snout bream (♀) × Bleeker’s yellow tail (Xenocypris davidi Bleeker, ♂) has showed significantly higher growth rate compared to their parents [13]. Hybridization can lead to rapid genomic changes, including chromosomal rearrangements, genome expansion, genomic DNA variation, differential gene expression, and gene silencing [14]. One such example is that of Brassica hybrids, in which multiple genome rearrangements and segment deletions occurred within five generations [15]. In addition, Rieseberg et al. found extensive genomic reorganization and karyotypic evolution in Helianthus hybrids, indicating the occurrence of rapid karyotypic evolution [16]. In Cyprinidae, in the allotetraploid hybrids, chimeric genes (9.67–11.06%) and mutation events (1.02–1.16%) occurred in different generations of this nascent allopolyploids [17]; Liu et al. revealed 19.04%, 4.17% chimeric genes and 6.90%, 5.05% mutations of orthologous genes in F1 and F2 of diploid hybrids, respectively [18]. Distant hybridization can generate changes in phenotypes and genotypes, leading to the formation of new hybrid lineages with genetic variation and providing a good experimental model for tracing the changes of genetic and epigenetic levels in the early stage of distant hybridization. Moreover, these newly established bisexual fertile diploid and tetraploid lineages provide new germplasm resources, which are used to produce improved diploid and triploid varieties by crossing diploid species, respectively [19,20,21,22].
In our previous study, we successfully obtained two types of improved carp offspring from common carp (2n = 100, abbreviated COC) (♀) × blunt snout bream (2n = 48, abbreviated BSB) (♂); one is the improved diploid common carp (2n = 100, IDC-F1), and the other is the improved diploid scattered mirror carp (2n = 100, IDMC-F1) [23]. In this study, we carried out self-crossing of these two types of improved carp offspring (IDC-F1 and IDMC-F1), respectively. Interestingly, the self-crossed offspring of IDC-F1 showed two phenotypes: one was consistent with that of their parents (abbreviated IDC-F2-C), and the other was very similar to that of IDMC-F1 (abbreviated IDC-F2-M) (Fig. 1a). In contrast, the self-crossed offspring of IDMC-F1 showed only one phenotype, that is, a scattered mirror carp-like appearance (abbreviated IDMC-F2) (Fig. 1a). To further explore the relationship of the genetic evolution of COC, IDC and IDMC, we studied the Hox gene structures in the genomic DNA of the different generations of the IDC and IDMC lineages. Determination of the genotypes of these lineages is very useful for understanding the processes associated with the genomic DNA changes that accompany phenotype changes.
Crossing procedure and appearances of COC, BSB, IDC-F1, IDMC-F1, IDC-F2-C, IDC-F2-M, and IDMC-F2; variable sequence types (including haplotypes and recombinant clusters) in different Hox genes in these species. a Crossing procedure and appearances of COC, BSB, IDC-F1, IDMC-F1, IDC-F2-C, IDC-F2-M, and IDMC-F2. b Variable sequence types (including haplotypes and recombinant clusters) in HoxA4a in these species. c Variable sequence types (including haplotypes and recombinant clusters) in HoxD4a in these species. d Variable sequence types (including haplotypes and recombinant clusters) in HoxD10a in these species
Hox genes, which encode transcription factors, are essential for the development of various morphological features. In vertebrates, Hox genes consist of two exons and the highly conserved homeodomain (60 aa), which is encoded by the second exon [24]. Late evolutionary novelties are generally considered to be associated either with the emergence of particular lineages or with important steps in their unique evolution [25]. Recent studies have shown that the origin and evolution of the Hox genes played a crucial role in genome replication, sequence variation, and selective pressure [25,26,27,28]. The search for regulatory elements through comparative genomic approaches using Hox genes promises to be particularly successful because their nucleotide sequences and functions are extremely conserved in all vertebrates; meanwhile, Hox gene clusters provide a good starting point for the study of genetic variation in genomic DNA [29].
Results
Sequence information for COC, BSB, IDC-F1, IDMC-F1, IDC-F2-C, IDC-F2-M, and IDMC-F2 clones
In this study, we used 12 pairs of degenerate PCR primers (Additional file 1: Table S1) to obtain partial sequence information for 20 putative Hox genes from COC, 12 putative Hox genes from BSB, 42 putative and 15 recombinant Hox genes from IDC-F1, 19 putative and 5 recombinant Hox genes from IDMC-F1, 17 putative and 12 recombinant Hox genes from IDMC-F2, 19 putative and 10 recombinant Hox genes from IDC-F2-C, and 18 putative and 13 recombinant Hox genes from IDC-F2-M. All of these fragments were between 700 and 1600 bp in length, including the exon 1-intron-exon 2 region (Tables 1 and 2). In this study, to avoid biased amplification of only one copy of the characterized Hox genes, we selected 30 clones of each gene from IDC-F1, IDMC-F1, IDC-F2-C, IDC-F2-M, and IDMC-F2 and 20 clones of each gene from COC and BSB. All fragments from COC, BSB, IDC-F1, IDMC-F1, IDC-F2-C, IDC-F2-M, and IDMC-F2 were confirmed to be Hox gene sequences via the NCBI website (http://www.ncbi.nlm.nih.gov), and each included the conserved homeobox region. All of the sequence information and GenBank accession numbers in this study is detailed in Additional file 1: Table S2.
Molecular organization of the Hox genes sequences
The organization of the Hox clusters in COC, BSB, IDC-F1, IDMC-F1, IDC-F2-C, IDC-F2-M, and IDMC-F2 are shown in Tables 1 and 2. Figure 1 and Additional file 2: Figures S1-S3 visually reflect the genetic variation in the Hox gene clusters of the two types of improved carp lineages. The Hox gene cluster organization showed that, as the first generation of distant hybridization, IDC-F1 had undergone extremely significant mutations; for example, in HoxA4a, IDC-F1 has five putative clusters and seven recombinant clusters (Fig. 1b and Tables 1 and 2); in HoxD4a, IDC-F1 has six putative clusters and one recombinant cluster (Fig. 1c and Tables 1 and 2); in HoxD10a, IDC-F1 has five putative clusters and two recombinant clusters (Fig. 1d and Tables 1 and 2). However, most of the Hox gene clusters in IDC-F1 (with a total of 42 putative and 15 recombinant Hox gene clusters) were not stably inherited in the second generation (IDC-F2-C and IDC-F2-M): IDC-F2-C has only 19 putative and 10 recombinant Hox gene clusters (Fig. 1, Additional file 2: Figures S1-S3 and Tables 1 and 2), and IDC-F2-M has only 18 putative and 13 recombinant Hox gene clusters (Fig. 1, Additional file 2: Figures S1-S3 and Tables 1 and 2). Additionally, although it was the first generation of a distant hybridization, we did not find obvious mutations in the Hox genes of the first generation of IDMC; almost all of the Hox gene clusters were derived from the female parent, COC, except for five recombinant clusters (Fig. 1, Additional file 2: Figures S1-S3 and Tables 1 and 2). Almost all of the Hox gene clusters in IDMC-F1 were stably inherited by the second generation (IDMC-F2), but IDMC-F2 had more obvious recombination events (the number of recombinant clusters increased to 12) (Fig. 1, Additional file 2: Figures S1-S3 and Tables 1 and 2). In this study, the self-crossed offspring of IDC-F1 showed two phenotypes. One of the offspring, IDC-F2-M, had a similar phenotype to that of IDMC-F1, so we searched for similarities and differences among the Hox gene clusters between the offspring IDC-F2-M and IDMC-F1 or IDMC-F2. Notably, we found similarities in the Hox gene clusters of these species (Fig. 1, Additional file 2: Figures S1-S3 and Tables 1 and 2); for example, the type of recombinant cluster including HoxA11b (HoxA11bi + HoxA11b-BSB + HoxA11bi) was found in only IDC-F2-M and IDMC-F2 (Additional file 2: Figure S1 c). In addition, as shown in Fig. 1, Additional file 2: Figures S1-S3 and Tables 1 and 2, IDC-F2-M possessed more abundant Hox gene clusters than IDMC-F1, similar to IDMC-F2, except that the Hox genes of IDMC-F2 were mainly concentrated in recombinant clusters. Among these Hox gene clusters, we found that all copies of HoxB4a in COC, BSB, IDC-F1, IDMC-F1, IDC-F2-C, IDC-F2-M, and IDMC-F2 were pseudogenes containing a stop codon that prematurely terminates the expression of a full-length functional product (Fig. 2a, b and Tables 1 and 2). We also found that the copies of HoxB1ai in COC, IDC-F1, IDMC-F1, IDC-F2-C, IDC-F2-M, and IDMC-F2 were pseudogenes due to stop codons (Fig. 2c, d and Tables 1 and 2). These results revealed that the Hox gene family in cyprinid fishes had undergone rapid evolution, with some genes gradually becoming pseudogenes, and some genes completely pseudogenised. Moreover, we also found pseudogenes in the recombinant clusters; for example, HoxB1ai + HoxB1aii and HoxB1aii + HoxB1ai in IDC-F2-M (Fig. 2c and Tables 1 and 2) and HoxC4aii + HoxC4ai in IDMC-F1 (Fig. 2e and Tables 1 and 2).
Pseudogene sequences of HoxB1a, HoxB4a, and HoxC4a. a The nucleotide sequences of HoxB4a in COC, BSB, IDC-F1, IDMC-F1, IDC-F2-C, IDC-F2-M, and IDMC-F2. b The putative amino acid sequence of HoxB4ai in COC. c The nucleotide sequences of HoxB1a in COC, BSB, IDC-F1, IDMC-F1, IDC-F2-C, IDC-F2-M, and IDMC-F2. d The putative amino acid sequence of HoxB1ai in COC. e The nucleotide sequences of HoxC4a in COC and IDMC-F1. The red boxes indicate the stop codon bases. The green boxes indicate that there is no corresponding amino acid site due to the occurrence of the stop codon, and the “*” sign is used instead
Phylogenetic relationships
An unrooted phylogenetic tree of 12 Hox genes was constructed using MrBayes based on the alignment results (Fig. 3). The overall phylogenetic tree was divided into twelve well-conserved clades, and each clade contained one zebrafish Hox gene. Meanwhile, we analysed the percentage nucleotide identity and the percentage amino acid identity between duplicated Hox coding regions in COC, BSB, IDC-F1, IDMC-F1, IDMC-F2, IDC-F2-C, and IDC-F2-M (Tables 3 and 4). As shown in Tables 3 and 4, the close relationships were observed among IDC-F1, IDC-F2-C, and IDC-F2-M within the IDC lineage; between IDMC-F1 and IDMC-F2 within the IDMC lineage; and among IDC-F1, IDMC-F1, IDMC-F2, IDC-F2-C, and IDC-F2-M within both lineages. To evaluate the speciation of the two types of improved carp lineages, the percentages of nucleotide (amino acid) identity among the 12 Hox gene groups in COC, BSB, and both improved carp lineages were examined (Tables 3 and 4, Fig. 3). The identities of the orthologous Hox genes between the two types of improved carp lineages and COC were much higher than those between the two types of improved carp lineages and BSB, except for the gene clusters inherited from BSB. In some Hox genes, such as HoxA4a, HoxA2b and HoxC4a, both the nucleotide and amino acid sequences of both improved carp lineages had a high degree of identity to COC and BSB. In some Hox genes, such as HoxA11b, HoxC6b, HoxD4a and HoxD10a, although the nucleotide sequences between the two types of improved carp lineages and COC or BSB had lower identities, they had higher amino acid sequence identities, which suggested that most mutations were synonymous. In some Hox genes, such as HoxA9a, both the nucleotide and amino acid sequences of both improved carp lineages had a low degree of identity to COC and BSB (Tables 3 and 4).
Phylogenetic analyses of the amino acid sequences of 12 Hox genes (HoxA4a, HoxA9a, HoxA2b, HoxA11b, HoxB1a, HoxB4a, HoxB1b, HoxB5b, HoxC4a, HoxC6b, HoxD4a, and HoxD10a) in COC, BSB, IDC-F1, IDMC-F1, IDC-F2-C, IDC-F2-M, IDMC-F2 and zebrafish (Danio rerio). Phylogenetic tree constructed using MrBayes with the HKY + I + G model (−lnL = 15,356.5967); MCMC = 2 million generations. The phylogenetic tree for each Hox gene is marked by a separate colour, as shown in the figure
Discussion
Hybridization offers a means by which diversity may be increased because, unlike mutation, it provides genetic variation at hundreds or thousands of genes in a single generation [4]. Our results provide a good model for genetic variation by showing obvious genotypic differences in the IDC-F1 fish derived from the distant hybridization of COC (♀) × BSB (♂). The Hox gene clusters in IDC-F1 were approximately twice as large as those in COC, except for the recombinant clusters. The topology of the phylogenetic tree of 12 Hox genes (Fig. 3) further suggested that some of the Hox genes orthologous to zebrafish genes were present as two copies in COC (except for HoxA11b, HoxB1b, HoxB4a, and HoxC6b), one copy in BSB, and two to six copies (not counting recombinant clusters) in IDC-F1. The proliferation of such a rich diversity in gene copy number further reveals that distant hybridization as a catalyst accelerates the formation of species [8]. One of the highlights of this study is the development of IDMC-F1 derived from the distant hybridization of COC (♀) × BSB (♂), which has a significant difference in phenotype compared to its parents; even in the self-crossed offspring of IDC-F1, two distinct phenotypes were differentiated: IDC-F2-C was consistent with that of IDC-F1, and IDC-F2-M was very similar to that of IDMC-F1 (Fig. 1). Determining the mechanisms that lead to these new phenotypes to appear will help us to understand the impact of hybridization on the speciation processes. At present, three possible mechanisms are considered. Firstly, alleles of additive effect may not all be fixed in the same direction between diverging populations, under this mechanism, some hybrid genotypes then fall outside the parental distribution (+ + + − × − − − + can generate + + + + or − − − −) [30]. Secondly, these new phenotypes derived from hybridization may result from interactions (dominance or epistasis) between alleles fixed independently in different populations. Thirdly, research in recent years has begun to reveal a wider variety of genetic mechanisms underlying new hybrid phenotypes, e.g., genome restructuring, duplication/deletion [31], alterations in the timing and levels of gene expression, transposon activation and epigenetic effects [32,33,34,35]. We speculated that the third mechanism was the possible reason for the differentiation of the mirror carp-type offspring (IDMC-F1 and IDC-F2-M). Under this mechanism, the genomes of hybrid progeny contain a rich variety of genetic variants, which are rapidly changing in the early generation of hybridization, and most of the variant types cannot be stably inherited to the next generation. In fact, most of the Hox gene copies in IDC-F1 were not stably inherited in the second generation (IDC-F2-C and IDC-F2-M). These result validated the possible mechanism of the differentiation of the mirror carp-type offspring. In contrast, as with the first generation of distant hybridization, we did not find obvious mutations in Hox genes in the first generation of IDMC; almost all of the Hox gene clusters were derived from the female parent, COC, except for the recombinant clusters. Almost all of the Hox gene clusters in IDMC-F1 were stably inherited in IDMC-F2, but at the same time, IDMC-F2 contained more obvious recombination events. The gene types that were stably inherited from a single parent in the offspring have experienced long-term evolutionary testing and became essential for the evolution of species.
The functions of Hox genes have become increasingly clear in recent years, but questions about the evolution of Hox genes remain unresolved. Gene duplication and mutation are the basis for understanding Hox gene evolution, and mutations in coding sequences may produce new functional proteins. Hox gene clusters in fish are more variable in gene content than expected, and each cluster has its own characteristics in terms of absolute length and content of conserved non-coding sequences [36]. This study fully confirms this argument; for example, among these Hox genes, two to six copies (not counting recombinant clusters) were found in IDC-F1. Furthermore, Hox cluster degeneration may be ongoing, at least in fish, because HoxB4a is active in zebrafish but its orthologues are pseudogenes in COC, BSB, IDC-F1, IDMC-F1, IDC-F2-C, IDC-F2-M, and IDMC-F2. Similarly, HoxB1ai is active in zebrafish, but its orthologues are pseudogenes in COC, IDC-F1, IDMC-F1, IDC-F2-C, IDC-F2-M, and IDMC-F2. These results revealed that the Hox gene clusters are undergoing continuous degeneration in the cyprinid fishes, with some genes gradually becoming pseudogenes, and some genes completely pseudogenised.
One of the most important finding of this study is the discovery of Hox gene recombinant clusters, which may be the first in Hox genes of cyprinid fishes or even vertebrates. In the two types of improved carp lineages derived from COC (♀) × BSB (♂), these recombinant clusters come from the recombination of different types of gene copies, most of which cannot be stably inherited to the next generation. Moreover, for HoxA11b, we found the recombinant cluster type (HoxA11bi + HoxA11b-BSB + HoxA11bi) only in IDC-F2-M and IDMC-F2, indicating that it might be necessary for development of the morphological features of mirror carp-like species. In this study, we studied the genetic variation in 12 Hox genes in the two types of improved carp lineages derived from COC (♀) × BSB (♂). We first revealed the interesting results of the abundant gene clusters derived from IDC-F1 and found a wide variety of recombinant clusters in the two types of improved carp lineages. In summary, our results provided important evidence that distant hybridization produced rapid genomic DNA changes that may or may not stably inherited, providing novel insight into the function of hybridization in the establishment of the improved lineages used as new fish resources for aquaculture. The genetic evolution of the Hox gene family provides clues for revealing the gene regulatory mechanisms underlying biological evolution and cell differentiation.
Conclusions
Based on the establishment of the two types of improved carp lineages derived from common carp (♀) × blunt snout bream (♂), our results provided important evidence that distant hybridization produced rapid genomic DNA changes that may or may not stably inherited, providing novel insight into the function of hybridization in the establishment of the improved lineages used as new fish resources for aquaculture.
Methods
Ethics statement
The guidelines established by the Administration of Affairs Concerning Animal Experimentation state that approval from the Science and Technology Bureau of China and the Department of Wildlife Administration is not necessary when the fish in question are neither rare nor near extinction (first- or second-class state protection level). Therefore, approval was not required for the experiments conducted in this study.
Animals and crossing procedure
All of the natural materials, such as common carp (Cyprinus carpio, 2n = 100, abbreviated as COC) and blunt snout bream (Megalobrama amblycephala, 2n = 48, abbreviated as BSB) were obtained from the Center for Polyploidy Fish Genetics Breeding of Hunan Province located at Hunan Normal University, Changsha, Hunan, China. The protocols for crossing and culturing were described previously [23]. The two types of improved carp offspring from COC (♀) × BSB (♂) were the improved diploid common carp (2n = 100, IDC-F1) and the improved diploid scattered mirror carp (2n = 100, IDMC-F1); the phenotype of the latter has changed significantly from that of the female parent, COC. The self-crossed offspring of IDC-F1 showed two phenotypes: one was consistent with that of their parents (abbreviated IDC-F2-C), and the other was very similar to that of IDMC-F1 (abbreviated IDC-F2-M). In contrast, the self-crossed offspring of IDMC-F1 showed only one phenotype, that is, a scattered mirror carp-like appearance (abbreviated IDMC-F2). The IDC-F1, IDMC-F1, IDC-F2-C, IDC-F2-M, and IDMC-F2 fish were cultured in ponds at the Center for Polyploidy Fish Genetics Breeding of Hunan Province located at Hunan Normal University, Changsha, Hunan, China, and fed artificial feed. All fishes were deeply anaesthetized with 100 mg/L MS-222 (Sigma-Aldrich, St. Louis, MO, USA) prior to dissection.
DNA extraction, PCR amplification, cloning and sequencing of Hox genes
Total genomic DNA from the peripheral blood cells of COC, BSB, IDC-F1, IDMC-F1, IDC-F2-C, IDC-F2-M, and IDMC-F2 extracted by routine approaches [37] were used separately as templates. Several combinations of degenerate PCR primers (Additional file 1: Table S1) [38, 39] were used to amplify up to 12 Hox gene sequences (HoxA4a, HoxA9a, HoxA2b, HoxA11b, HoxB1a, HoxB4a, HoxB1b, HoxB5b, HoxC4a, HoxC6b, HoxD4a, and HoxD10a) in COC, BSB, IDC-F1, IDMC-F1, IDC-F2-C, IDC-F2-M, and IDMC-F2. The PCRs were performed in a volume of 50 μL using Taq DNA polymerase (TaKaRa, Dalian, China). The thermal cycling program uses thermal gradient PCR and used these conditions for the first time. The thermal cycling program generally consisted of an initial denaturation step at 94 °C for 5 min, followed by 35 cycles of 94 °C for 35 s, 50–60 °C for 60 s, and 72 °C for 60–150 s and a final extension step at 72 °C for 10 min. The PCR products were cloned into the pMD18-T vector (TaKaRa, Dalian, China). The plasmids were transformed into E. coli DH5a, purified and sequenced with vector-specific primers using the primer walking method on an ABI 3730XL automatic sequencer (ABI PRISM 3730, Applied Biosystems, CA, USA). The sequences were BLAST searched against the non-redundant protein database maintained at the National Center for Biotechnology Information (www.ncbi.nlm.nih.gov) to determine their identity.
Sequence comparison and analysis
All of the sequence information and GenBank accession numbers in this study is detailed in Additional file 1: Table S2. The sequence homology and variation among the fragments amplified from COC, BSB, IDC-F1, IDMC-F1, IDC-F2-C, IDC-F2-M, and IDMC-F2 were analysed using BioEdit [40] and the DNAStar 5.0 software package (DNAStar Inc.). To increase the probability of detecting duplicated paralogs and circumventing errors from PCR, we sequenced 20–30 clones for each gene from each of COC, BSB, IDC-F1, IDMC-F1, IDC-F2-C, IDC-F2-M, and IDMC-F2. The obtained sequences were screened for Hox gene fragments using the BLAST (http://www.ncbi.nlm.nih.gov), ClustalW (http://www.ebi.ac.uk/) [41] and MEGA 4.0 [42] programs to determine identity. Then, we evaluated the organization of the Hox clusters in IDC-F1, IDMC-F1, IDC-F2-C, IDC-F2-M, and IDMC-F2 in comparison with COC and BSB to characterize the Hox genes.
Phylogenetic analysis - unconstrained Bayesian analysis
The derived amino acid sequences of 12 Hox genes (HoxA4a, HoxA9a, HoxA2b, HoxA11b, HoxB1a, HoxB4a, HoxB1b, HoxB5b, HoxC4a, HoxC6b, HoxD4a, and HoxD10a) in COC, BSB, IDC-F1, IDMC-F1, IDC-F2-C, IDC-F2-M, IDMC-F2 were aligned with the Hox genes of zebrafish retrieved from GenBank using Clustal X 1.81 [43]. Regions of zebrafish Hox gene sequence that were difficult to align were removed from the alignment. Gaps were also removed from the alignment. An unrooted phylogenetic tree of all amino acid sequences of the 12 Hox genes (the pseudogenes found in this study were not excluded from the phylogenetic analysis) was analysed in MrBayes version 3.1.2 [44, 45]. We also tested the Hox genes for saturation using DAMBE v6.4.41 [46], and the results revealed that the Hox genes were suitable for phylogenetic analysis. The best-fitting substitution models for each gene fragment were determined by Modeltest 3.7 [47], and the HKY + I + G model was chosen for the Hox genes by using the Bayesian information criterion. MrBayes was run for 2 million generations with two runs and four chains in parallel and a burn-in of 25%, and the analysis was terminated after the average standard deviation of the split frequencies fell under 0.01. The final trees were visualized in FIGTREE 1.4.4 (http://tree.bio.ed.ac.uk/software/figtree/. 2018.).
Availability of data and materials
The sequencing data are deposited in NCBI (http://www.ncbi.nlm/.nih.gov/nuccore) and is available from the authors. All of the sequence information and GenBank accession numbers in this study is detailed in Additional file 1: Table S2. The fish cannot be made publicly available, because they are property of the Hunan Normal University.
Abbreviations
- BSB:
-
Blunt snout bream (Megalobrama amblycephala)
- COC:
-
Common carp (Cyprinus carpio)
- IDC-F1 :
-
The first generation of the improved diploid common carp derived from common carp (♀) × blunt snout bream (♂)
- IDC-F2-C:
-
The self-crossed offspring of IDC-F1 (common carp-type)
- IDC-F2-M:
-
The self-crossed offspring of IDC-F1 (mirror carp-type)
- IDMC-F1 :
-
The first generation of the improved diploid scattered mirror carp derived from common carp (♀) × blunt snout bream (♂)
- IDMC-F2 :
-
The self-crossed offspring of IDMC-F1
References
Abbott R, Albach D, Ansell S, Arntzen JW, Baird SJ, Bierne N, Boughman J, Brelsford A, Buerkle CA, Buggs R, et al. Hybridization and speciation. J Evol Biol. 2013;26(2):229–46.
Mallet J. Hybridization as an invasion of the genome. Trends Ecol Evol. 2005;20(5):229–37.
Grant PR, Grant BR. Phenotypic and genetic effects of hybridization in Darwin's finches. Evolution. 1994;48(2):297–316.
Rieseberg LH, Raymond O, Rosenthal DM, Lai Z, Livingstone K, Nakazato T, Durphy JL, Schwarzbach AE, Donovan LA, Lexer C. Major ecological transitions in wild sunflowers facilitated by hybridization. Science. 2003;301(5637):1211–6.
Arnold ML, Martin NH. Adaptation by introgression. J Biol. 2009;8(9):82.
Whitney KD, Randell RA, Rieseberg LH. Adaptive introgression of abiotic tolerance traits in the sunflower Helianthus annuus. New Phytol. 2010;187(1):230–9.
Kunte K, Shea C, Aardema ML, Scriber JM, Juenger TE, Gilbert LE, Kronforst MR. Sex chromosome mosaicism and hybrid speciation among tiger swallowtail butterflies. PLoS Genet. 2011;7(9):e1002274.
Mallet J. Hybrid speciation. Nature. 2007;446(7133):279–83.
Stukenbrock EH. Hybridization speeds up the emergence and evolution of a new pathogen species. Nat Genet. 2016;48(2):113–5.
Huang ML, Deng XP, Zhao YZ, Zhou SL, Inanaga S, Yamada S, Tanaka K. Water and nutrient use efficiency in diploid, tetraploid and hexaploid wheats. J Integr Plant Biol. 2007;49(5):706–15.
Takahashi Y, Iseki K, Kitazawa K, Muto C, Somta P, Irie K, Naito K, Tomooka N. A Homoploid hybrid between wild Vigna species found in a limestone karst. Front Plant Sci. 2015;6.
Qin Q, Wang Y, Wang J, Dai J, Xiao J, Hu F, Luo K, Tao M, Zhang C, Liu Y, et al. The Autotetraploid fish derived from hybridization of Carassius auratus red var. (female) x Megalobrama amblycephala (male). Biol Reprod. 2014;91(4):93.
Hu J, Liu S, Xiao J, Zhou Y, You C, He W, Zhao R, Song C, Liu Y. Characteristics of diploid and triploid hybrids derived from female Megalobrama amblycephala Yih×male Xenocypris davidi Bleeker. Aquaculture. 2012;364-365:157–64.
Baack EJ, Rieseberg LH. A genomic view of introgression and hybrid speciation. Curr Opin Genet Dev. 2007;17(6):513–8.
Song K, Lu P, Tang K, Osborn TC. Rapid genome change in synthetic polyploids of Brassica and its implications for polyploid evolution. Proc Natl Acad Sci. 1995;92(17):7719–23.
Rieseberg LH, Van Fossen C, Desrochers AM. Hybrid speciation accompanied by genomic reorganization in wild sunflowers. Nature. 1995;375(6529):313–6.
Liu S, Luo J, Chai J, Ren L, Zhou Y, Huang F, Liu X, Chen Y, Zhang C, Tao M. Genomic incompatibilities in the diploid and tetraploid offspring of the goldfish× common carp cross. Proc Natl Acad Sci. 2016;201512955.
Liu Q, Qi Y, Liang Q, Xu X, Hu F, Wang J, Xiao J, Wang S, Li W, Tao M. The chimeric genes in the hybrid lineage of Carassius auratus cuvieri (♀)× Carassius auratus red var.(♂). Sci China Life Sci. 2018:1–11.
Liu S. Distant hybridization leads to different ploidy fishes. Sci China Life Sci. 2010;53(4):416–25.
Song C, Liu S, Xiao J, He W, Zhou Y, Qin Q, Zhang C, Liu Y. Polyploid organisms. Sci China Life Sci. 2012;55(4):301–11.
Zhang Z, Chen J, Li L, Tao M, Zhang C, Qin Q, Xiao J, Liu Y, Liu S. Research advances in animal distant hybridization. Sci China Life Sci. 2014;57(9):889–902.
Wang S, Tang C, Tao M, Qin Q, Zhang C, Luo K, Zhao R, Wang J, Ren L, Xiao J, et al. Establishment and application of distant hybridization technology in fish. Sci China Life Sci. 2019;62(1):22–45.
Wang S, Ye X, Wang Y, Chen Y, Lin B, Yi Z, Mao Z, Hu F, Zhao R, Wang J, et al. A new type of homodiploid fish derived from the interspecific hybridization of female common carp x male blunt snout bream. Sci Rep. 2017;7(1):4189.
Koh EG, Lam K, Christoffels A, Erdmann MV, Brenner S, Venkatesh B. Hox gene clusters in the Indonesian coelacanth, Latimeria menadoensis. Proc Natl Acad Sci U S A. 2003;100(3):1084–8.
Duboule D. The rise and fall of Hox gene clusters. Development. 2007;134(14):2549–60.
Meyer A, Van de Peer Y. From 2R to 3R: evidence for a fish-specific genome duplication (FSGD). Bioessays. 2005;27(9):937–45.
Crow KD, Stadler PF, Lynch VJ, Amemiya C, Wagner GP. The "fish-specific" Hox cluster duplication is coincident with the origin of teleosts. Mol Biol Evol. 2006;23(1):121–36.
Lemons D, McGinnis W. Genomic evolution of Hox gene clusters. Science. 2006;313(5795):1918–22.
Doerks T, Copley RR, Schultz J, Ponting CP, Bork P. Systematic identification of novel protein domain families associated with nuclear functions. Genome Res. 2002;12(1):47–56.
Orr HA. Testing natural selection vs. genetic drift in phenotypic evolution using quantitative trait locus data. Genetics. 1998;149(4):2099–104.
Nei M, Nozawa M. Roles of mutation and selection in speciation: from Hugo de Vries to the modern genomic era. Genome Biol Evol. 2011;3:812–29.
Landry C, Hartl D, Ranz J. Genome clashes in hybrids: insights from gene expression. Heredity. 2007;99(5):483.
Doyle JJ, Flagel LE, Paterson AH, Rapp RA, Soltis DE, Soltis PS, Wendel JF. Evolutionary genetics of genome merger and doubling in plants. Annu Rev Genet. 2008;42:443–61.
Michalak P. Epigenetic, transposon and small RNA determinants of hybrid dysfunctions. Heredity. 2009;102(1):45–50.
Ainouche ML, Jenczewski E. Focus on polyploidy. New Phytol. 2010;186(1):1–4.
Hoegg S, Boore JL, Kuehl JV, Meyer A. Comparative phylogenomic analyses of teleost fish Hox gene clusters: lessons from the cichlid fish Astatotilapia burtoni. BMC Genomics. 2007;8:317.
Sambrook J, Russell David W: Molecular cloning: a laboratory manual. Vol. 3: Cold spring harbor laboratory press; 1989.
Luo J, Stadler PF, He S, Meyer A. PCR survey of hox genes in the goldfish Carassius auratus auratus. J Exp Zool B Mol Dev Evol. 2007;308(3):250–8.
Wang YD, Qin QB, Yang R, Sun WZ, Liu QW, Huo YY, Huang X, Tao M, Zhang C, Li T, et al. Hox genes reveal genomic DNA variation in tetraploid hybrids derived from Carassius auratus red var. (female) x Megalobrama amblycephala (male). BMC Genet. 2017;18(1):86.
Hall TA: BioEdit: a user-friendly biological sequence alignment editor and analysis program for windows 95/98/NT. In: Nucleic acids symposium series: 1999. 95–98.
Thompson JD, Higgins DG, Gibson TJ. CLUSTAL W: improving the sensitivity of progressive multiple sequence alignment through sequence weighting, position-specific gap penalties and weight matrix choice. Nucleic Acids Res. 1994;22(22):4673–80.
Tamura K, Dudley J, Nei M, Kumar S. MEGA4: molecular evolutionary genetics analysis (MEGA) software version 4.0. Mol Biol Evol. 2007;24(8):1596–9.
Thompson JD, Gibson TJ, Plewniak F, Jeanmougin F, Higgins DG. The ClustalX windows interface: flexible strategies for multiple sequence alignment aided by quality analysis tools. Nucleic acids res. Nucleic Acids Res. 1997;25(24):4876–82.
Huelsenbeck JP, Ronquist F. MRBAYES: Bayesian inference of phylogenetic trees. Bioinformatics. 2001;17(8):754–5.
Ronquist F, Huelsenbeck JP. MrBayes 3: Bayesian phylogenetic inference under mixed models. Bioinformatics. 2003;19(12):1572–4.
Xia X. DAMBE5: a comprehensive software package for data analysis in molecular biology and evolution. Mol Biol Evol. 2013;30(7):1720–8.
Posada D, Crandall KA. Modeltest: testing the model of DNA substitution. Bioinformatics (Oxford, England). 1998;14(9):817–8.
Acknowledgements
We would like to sincerely thank many researchers who helped to complete this manuscript, including Rurong Zhao, Zhanzhou Yao, Qingfeng Liu and Liu Cao.
Funding
This work was supported by the National Natural Science Foundation of China (Grant Nos. 31802287, 31730098, 31760306, 31430088), Changsha Science and Technology Achievements Transformation Project (Grant No. kq1901087), the China Postdoctoral Science Foundation (Grant No. 2018 M632969), the earmarked fund for China Agriculture Research System (Grant No. CARS-45), the Key Research and Development Program of Hunan Province (Grants No. 2018NK2072), Hunan Provincial Natural Science and Technology Major Project (Grant No. 2017NK1031), and the Cooperative Innovation Center of Engineering and New Products for Developmental Biology of Hunan Province (Grant No. 20134486). The funding bodies play no role in the design of the study and collection, analysis and interpretation of data and in writing the manuscript.
Author information
Authors and Affiliations
Contributions
KKL, SW and SJL designed the study, performed the analyses, performed the technical discussions, and prepared and drafted the manuscript. YQF, PZ, XXH and QHG performed the experimental work and participated in data discussions. WHL, YDW and FZH were involved in the statistical analysis. All authors read and approved the final manuscript.
Corresponding author
Ethics declarations
Ethics approval
All experiments were approved by the Animal Care Committee of Hunan Normal University and followed the guidelines statement of the Administration of Affairs Concerning Animal Experimentation of China. All samples were raised in natural ponds, all dissections were performed under MS-222 (Sigma-Aldrich, St. Louis, MO, USA) anesthesia, and all efforts were made to minimize suffering.
This manuscript does not involve the use of any human data or tissue. So the consent to participate is not applicable.
Consent for publication
Not applicable.
Competing interests
The authors declare that they have no competing interests.
Additional information
Publisher’s Note
Springer Nature remains neutral with regard to jurisdictional claims in published maps and institutional affiliations.
Supplementary information
Additional file 1: Table S1.
The 12 combinations of degenerate PCR primers designed based on the alignment and identification of consensus sequences of orthologous Hox genes from zebrafish (Danio rerio), medaka (Oryzias latipes), rainbow trout (Oncorhynchus mykiss), pufferfish (Fugu rubripes), mouse (Mus musculus), and humans (Homo sapiens). Table S2. Sequence information and GenBank accession numbers for COC, BSB, IDC-F1, IDMC-F1, IDC-F2-C, IDC-F2-M, and IDMC-F2 clones, and GenBank accession numbers of zebrafish (Danio rerio) used in this study; the tick symbol means the suquence used in this analysis.
Additional file 2: Figure S1.
Variable sequence types (including haplotypes and recombinant clusters) in different Hox genes in these species. Figure S2. Variable sequence types (including haplotypes and recombinant clusters) in different Hox genes in these species. Figure S3. Variable sequence types (including haplotypes and recombinant clusters) in HoxB5b in these species.
Rights and permissions
Open Access This article is distributed under the terms of the Creative Commons Attribution 4.0 International License (http://creativecommons.org/licenses/by/4.0/), which permits unrestricted use, distribution, and reproduction in any medium, provided you give appropriate credit to the original author(s) and the source, provide a link to the Creative Commons license, and indicate if changes were made. The Creative Commons Public Domain Dedication waiver (http://creativecommons.org/publicdomain/zero/1.0/) applies to the data made available in this article, unless otherwise stated.
About this article
Cite this article
Luo, K., Wang, S., Fu, Y. et al. Rapid genomic DNA variation in newly hybridized carp lineages derived from Cyprinus carpio (♀) × Megalobrama amblycephala (♂). BMC Genet 20, 87 (2019). https://doi.org/10.1186/s12863-019-0784-2
Received:
Accepted:
Published:
DOI: https://doi.org/10.1186/s12863-019-0784-2