Abstract
Aluminum (Al) toxicity on acid soils adversely affects maize yields, which can be overcome by combining soil amendments with genetic tolerance. In maize, ZmMATE1 confers Al tolerance via Al-activated citrate release, whereby citrate forms non-toxic complexes with Al3+ in the rhizosphere. Here, we investigated Al tolerance mechanisms in maize germplasm originated from Kenya based on quantitative trait loci (QTL) mapping. Five QTLs and four epistatic interactions explained ~51% of the phenotypic variation for Al tolerance. The lack of Al tolerance QTL on chromosome 6 and the much lower expression of ZmMATE1 in both Kenyan lines than in Cateto Al237, which donates the superior allele of ZmMATE1, strongly indicate that this gene does not play a significant role in Al tolerance in neither parent. In turn, maize homologs to genes previously implicated in Al tolerance in other species, ZmNrat1, ZmMATE3, ZmWRKY and ZmART1, co-localized with Al tolerance QTL and were more highly expressed in the parent that donate favorable QTL alleles. However, these candidate genes will require further studies for functional validation on maize Al tolerance. The existence of Al tolerance mechanisms independent from ZmMATE1 suggests it is possible to develop highly Al tolerant cultivars by pyramiding complementary Al tolerance genes in maize.
Similar content being viewed by others
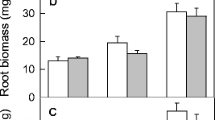
Introduction
Maize (Zea mays L.), the most produced cereal in the world, is largely used as animal feed, biofuel and is an important staple food in Africa and Latin America1. Maize occupies approximately 24% of all farmlands in Africa and accounts for 73% and 64% of the total food demand in Eastern-Southern and Western-Central Africa, respectively2. In Kenya, maize is consumed by approximately 96% of the population and is mainly produced on acidic soil regions where abiotic stresses contribute to very low maize yields (1.0–1.5 t/ha)3,4.
Maize yields are greatly reduced by Al toxicity on acid soils5, which cover approximately 50% of the global arable lands6, mainly in tropical and sub-tropical regions. Under pH below 5.5, the rhyzotoxic form of Al, Al3+, becomes prevalent in the soil solution, damaging plant root systems7,8,9. Hence, Al toxicity impairs soil exploitation, reducing water and nutrient uptake and, consequently, grain yield. The low soil pH problem can be ameliorated by lime application. However, liming is most effective in the arable soil layers10, whereas deeper soil correction is more difficult and costly. Moreover, soil amendments increase the production costs and are inaccessible for most small-hold farmers in developing countries11. Thus, a sustainable solution to enhance grain yields on acid soils can be achieved by associating soil fertility management practices with the adoption of Al tolerant cultivars.
Al tolerance in maize is a complex trait controlled by a few Quantitative Trait Loci (QTLs)12,13,14,15. The strongest maize Al tolerance QTL is located at bin 6.00 on chromosome 6, controlling 16 to 30% of the genetic variance in biparental populations derived from the highly Al-tolerant line, Cateto Al23715,16. Even though several genes have been identified controlling different mechanisms of Al tolerance in plants17, copy number variation of the Al-activated citrate transporter, ZmMATE1, was found to underlie the Al tolerance QTL on chromosome 616,18. ZmMATE1 expression is up-regulated by Al and three copies in tandem of the transporter gene were associated with enhanced ZmMATE1 expression and Al tolerance18. The superior allele of ZmMATE1 improving Al tolerance was donated by Cateto Al237 and was only found in two other lines, one from Brazil and another one from Bolivia18. Thus, the rare, favorable allele of ZmMATE1 is mainly present in maize lines from South America18.
Maize landraces and lines from Kenya also showed high Al tolerance in nutrient solution19,20, reaching levels similar to Cateto Al237, the Brazilian source of ZmMATE1. However, Kenyan maize genotypes showed a lower ZmMATE1 expression level at 6 hours after Al treatment than Cateto Al23721, suggesting the existence of different Al tolerance mechanisms in Kenyan germplasm. The present study aimed at identifying QTL and candidate genes associated with these mechanisms in an F2:3 population derived from the highly Al-tolerant Kenyan line, 203B-14, crossed with SCH3 (Al-sensitive).
Results
Variability of aluminum tolerance in the Kenyan maize population
Significant genotypic variation was detected for Al tolerance in F2:3 progeny derived from 203B-14 (Al tolerant) crossed with SCH3 (Al sensitive) based on relative net root growth (RNRG) (Supplementary Table S1). The heritability for Al tolerance based on family means was high (0.97), and the coefficient of experimental variation was low (8.82%) (Supplementary Table S1), indicating high quality of the phenotypic data.
The RNRG population mean was 35.2% (Supplementary Table S1) with minimum and maximum values of 17.6 and 68.3%, respectively (Fig. 1). The RNRG mean of the Al-tolerant parent 203B-14, 107.7%, was similar to the Brazilian Al-tolerant maize line, Cateto Al237 (95.2%), but higher than the most Al-tolerant F2:3 progeny (Fig. 1). On the other hand, the Al-sensitive parent, SCH3, was less sensitive to Al than the most Al-sensitive F2:3 progeny and the Brazilian Al-sensitive line L53 (Fig. 1).
Distribution of aluminum tolerance based on relative net root growth (RNRG %) in Kenyan maize progeny. The population was composed by 180 F2:3 progeny derived from SCH3 (Al-sensitive) × 203B-14 (Al-tolerant). RNRG means of Kenyan parents are shown by thick arrows, whereas that of the Brazilian standards for Al sensitivity and Al tolerance, L53 and Cateto Al237, respectively, are depicted by thin arrows.
Al tolerance QTLs
Out of the 198 markers genotyped in the population (183 SNPs, 14 SSRs and the ZmNrat1), 122 Mendelian markers (i.e. single locus segregation frequencies as expected for an F2 population) were used in the linkage map, covering 1,192.2 cM of the ten maize chromosomes (Fig. 2). Chromosome 1 had the highest number of markers (22) spanning 227.6 cM, whereas chromosome 7 had only seven markers along 87.1 cM. Four gaps ranging from 35 to 42 cM were present on chromosomes 2, 4, 7 and 10. The order of the markers flanking these gaps was confirmed by their physical positions according to MaizeGDB.
Aluminum tolerance QTLs in Kenyan maize population. The F2:3 203B-14 × SCH3 linkage map was composed by 122 markers distributed along the 10 maize chromosomes. QTLs are depicted by black lines on the right hand side of the map, whereas genetic distances based on the Kosambi function (in centiMorgans) are shown on the left.
Five Al tolerance QTLs were mapped to chromosomes 1, 5, 8, 9 and 10, individually explaining up to 5.5% of the phenotypic variance for RNRG (Table 1). A model with QTL main effects and four epistatic interactions explained 50.94% of the phenotypic variance for Al tolerance. The Al-tolerant parent (A) donated alleles increasing Al tolerance for three QTLs (qALT1.09, qALT5.03 and qALT10.02; Table 1 and Supplementary Fig. S1a), whereas the sensitive parent allele (B) increased Al tolerance for qALT8.05 and qALT9.01 (Table 1 and Supplementary Fig. S1b). For qALT1.09, qALT8.05 and qALT9.01, heterozygous progeny had lower RNRG than the mean of both homozygous classes (Fig. S1a,b), which resulted in dominance effects with negative signs (Table 1). On the other hand, heterozygous progeny for qALT5.03 and qALT10.02 showed higher RNRG than the mean of homozygous progeny (Fig. S1a).
Epistatic interactions between QTLs explained from 3.3 to 7.0% of the phenotypic variance for RNRG (Table 1), which was similar to the individual QTL main effects. In both epistatic interactions involving qALT8.05 (qALT8.05 × qALT1.09 and qALT8.05 × qALT9.01), F2:3 progeny homozygous for the Al-sensitive (SCH3) allele at qALT8.05 showed the highest RNRG mean (blue dots, Fig. 3a,b). For the interaction qALT10.02 × qALT1.09, the most Al tolerant progeny were homozygous for alleles donated by the tolerant parent at both QTLs (red triangles and A, Fig. 3c). The interaction between qALT9.01 × qALT1.09 showed that most of the Al sensitive progeny carried the unfavorable allele at qALT9.01 (Fig. 3d).
Al tolerance (RNRG means and standard deviations) for F2:3 progeny showing combinations of alleles at pairs of epistatic QTLs. Progeny homozygous for the allele donated by the Al-sensitive parent, SCH3, are shown in B (blue dot), whereas progeny homozygous for the Al-tolerant parent, 203B-14, are shown in A (red triangle). Means for heterozygous (H) progeny are also shown (green square). Interaction between QTLs (a) qALT8.05 × qALT1.09, (b) qALT8.05 × qALT9.01, (c) qALT10.02 × qALT1.09 and (d) qALT9.01 × qALT1.09.
Expression pattern of ZmMATE1
The lack of an Al tolerance QTL at bin 6.00 suggests that ZmMATE1 does not play a role in Al tolerance in the 203B-14 x SCH3. Then, the time course expression of ZmMATE1 in these parents was compared to the Al-tolerant Cateto Al237 and the Al-sensitive L53. The expression of ZmMATE1 in the root tips of both Kenyan lines was much lower than in Cateto Al237, which donates the superior allele of ZmMATE1, and was even lower when compared to the Al-sensitive line from Brazil, L53 (Fig. 4). The time course expression of ZmMATE1 in the Brazilian maize lines was in agreement with Maron et al.16.
Expression profile of ZmMATE1 in the parental maize lines from Kenya 203B-14 (Al-tolerant) and SCH3 (Al-sensitive) as well as in Cateto Al237 (Al-tolerant) and L53 (Al-sensitive). RNA was isolated from root apices (1 cm) sampled from seven seedlings for each biological replicate after 0, 1, 6, 12 and 24 hours of cultivation without (−Al) and with (+Al) {39} µM Al3+ activity in nutrient solution. 203B-14 cultivated without Al at time 0 was used as reference. Error bars depict the standard deviation of three biological replicates.
Candidate genes co-localized with Al tolerance QTLs
The number of predicted genes within each Al tolerance QTL ranged from 69 to 447 (Table 2), which were described in the Supplementary Table S2. We selected one to two candidates within each QTL interval based on similarity with other genes previously characterized for Al tolerance in other species. No previously characterized Al tolerance genes were found within the qALT1.09 and qALT9.01 intervals, suggesting that new candidate genes underlie these QTLs.
The qALT5.03 interval extended from 59.3 to 74.6 Mb on chromosome 5, and harbored two candidate genes, GRMZM2G065154 at 71.7 Mb and GRMZM2G168747 at 74.6 Mb (Table 2). GRMZM2G065154 encodes a MATE member (ZmMATE3) that shared 21.9% of sequence identity with ZmMATE1 (Supplementary Table S3), and clustered together with other citrate transporters associated with Al tolerance in several plant species (Fig. 5a). GRMZM2G168747 (ZmNrat1) had 81.8% sequence identity with OsNrat1 (Fig. 5b and Supplementary Table S3), an Al3+ transporter involved in rice Al tolerance22.
Phylogenetic trees of maize proteins predicted within the Al tolerance QTLs. Phylogenetic trees were based on maximum likelihood using the predicted amino acid sequences of maize candidate genes located within the Al tolerance QTLs, maize predicted gene with sequence identity higher than 21.8% and genes controlling Al tolerance in other plant species. (a) MATE transporters from rice, sorghum, barley, wheat, Brachypodium, Arabidopsis and maize. (b) Nrat1 aluminum transporters from rice and maize. (c) WRKY transcriptional regulators of Al tolerance genes from sorghum, rice, Arabidopsis and maize. (d) Transcriptional factor containing C2H2 motifs from sorghum, wheat, Arabidopsis, rice and maize.
qALT8.05 spanned a genomic region ranging from 111.2 to 133.8 Mb on chromosome 8, where GRMZM2G034421, which encodes a WRKY protein, was found at 118.5 Mb. GRMZM2G034421, designated henceforth as ZmWRKY, had 80.1% sequence identity with SbWRKY1 (Fig. 5c and Supplementary Table S3), a sorghum transcription factor that regulates SbMATE expression23.
GRMZM2G068710 is located at 10.1 Mb on chromosome 10, which is coincident with the qALT10.02 interval (9.7–13.5 Mb). GRMZM2G068710 is 59.4% similar to OsART1 (Fig. 5d and Supplementary Table S3), a transcriptional regulator of Al tolerance genes in rice24, and is predicted to contain C2H2 zinc finger motif.
Expression pattern of candidate genes in the parental lines
Temporal expression of candidate genes co-localized with Al tolerance QTLs was assessed in the root tips of the Al-tolerant and Al-sensitive parents, 203B-14 and SCH3, respectively. ZmMATE3 was up-regulated in the Al-tolerant line after 24 hours of Al treatment, and was expressed at higher levels in the Al-tolerant line compared to the Al-sensitive parent (Fig. 6a). ZmNrat1 expression was induced by Al in the root tip of the Al-tolerant line after 6 hours, reaching a plateau between 12 and 24 hours of Al exposure (Fig. 6b). ZmWRKY expression was higher in the Al-sensitive than in the Al-tolerant line, and was induced by Al in the Al-sensitive parent (Fig. 6c). ZmART1 was more highly expressed in the Al-tolerant than in the Al-sensitive parent but was neither induced by Al nor differentially expressed in any time-point (Fig. 6d).
Expression profile of the candidate genes co-localized with Al tolerance QTLs. The contrasting parents are 203B-14 (Al-tolerant) and SCH3 (Al-sensitive) treated without (−Al) and with (+Al) {39} µM Al3+ activity in nutrient solution along 0, 1, 6, 12 and 24 hours (h). RNA was isolated from root apices (1 cm) sampled from seven seedlings for each biological replicate. Error bars depict the standard deviation of three biological replicates. (a) Relative expression of ZmMATE3, (b) ZmNrat1, (c) ZmWRKY1, and (d) ZmART1.
Discussion
Several landraces collected at maize growing areas in Kenya showed high levels of Al tolerance in nutrient solution, including 203B, which was originated from an acid soil region in Muranga County19. The Al tolerant parent, 203B-14, was derived from selfing the landrace 203B. The existence of genetic variability for Al tolerance in landraces adapted to local agro-ecological regions can be used for molecular genetics studies and for breeding purposes. In this context, highly contrasting Kenyan maize lines for Al tolerance were selected and crossed to generate an F2:3 population, which was used for QTL mapping.
The QTLs and QTL interactions detected in this Kenyan population explained approximately 51% of the total variance for Al tolerance, which was lower but comparable to a QTL mapping study in a Brazilian population derived from Cateto Al237 × L53 (63%)15. Al tolerance in Cateto Al237 and 203B-14, parents of both the Brazilian and the Kenyan populations, respectively, was similar (~100% RNRG), which makes these lines highly Al tolerant15,19,20,21,25. The slight bias of derived F2:3 progeny towards Al sensitivity could be explained, at least in part, by the presence of three epistatic interactions between QTLs. High Al tolerance was observed in progeny that are double homozygous for favorable alleles at each pair of interacting QTLs (Fig. 3a–c). Taking two unlinked QTL loci whose alleles are assorted randomly, only 1/16 of the progeny are expected to be double homozygous for the favorable alleles. Hence, very few progeny should be highly Al tolerant, whereas the majority would express from medium to low Al tolerance.
No Al tolerance QTL was mapped on chromosome 6 (bin 6.00), which harbored the Al tolerance gene, ZmMATE116, whose expression was much lower in 203B-14 and SCH3 than in Cateto Al237. Consistent with these results, both Kenyan parental lines showed lower ZmMATE1 expression 6 hours after Al stress compared to Cateto Al23721. As Al-induced citrate transporter expression in the root tips leads to ZmMATE1-mediated Al tolerance in Cateto Al23716,18, low ZmMATE1 expression in 203B-14 strongly supports the lack of this functional allele in 203B-14. Thus, the high Al tolerance in this Kenyan line is likely controlled to a large extent by mechanisms other than Al exclusion mediated by ZmMATE1. As other Al-tolerant maize genotypes from Kenya also showed low ZmMATE1 expression21,25, it is possible that functional ZmMATE1 alleles are not present in Kenyan maize germplasm.
We selected candidate genes co-localized with Al tolerance QTLs, which will be discussed in the light of QTL effects and gene expression profiles. The Al tolerance qALT5.03 explained 5.4% of the Al tolerance phenotype and two candidate genes ZmMATE3 (GRMZM2G065154) and ZmNrat1 (GRMZM2G168747) are located within this genomic region. A positive correlation between citrate exudation and Al tolerance (r = 0.51, P < 0.05) in 12 Kenyan maize accessions has been previously reported25, suggesting that Al exclusion mediated by citrate release takes place in Kenyan maize germplasm. Phylogenetic studies of maize MATE-like proteins grouped ZmMATE3 with other MATE members previously characterized as citrate transporters in different plant species (Fig. 4b and Guimaraes et al.15). Different to the early induction reported for ZmMATE1 in Cateto Al23716, ZmMATE3 was induced by Al after 24 hours in 203B-14, which donated the qALT5.03 allele improving Al tolerance. As ZmMATE1 was not functional in this population, ZmMATE3 could provide such Al tolerance mechanism to 203B-14.
ZmNrat1 is 83% similar to OsNrat1, a plasma membrane Al3+ transporter required for Al detoxification in rice22. A reduction of OsNrat1 expression in rice resulted in shorter roots with higher Al concentration in the cell wall of root tips of the Al-sensitive parent compared to the Al-tolerant parent under Al stress26. In addition, OsNrat1 was found to underlie the Al tolerance QTL on rice chromosome 222,26. ZmNrat1 (GRMZM2G168747), which was also co-localized with qALT5.03, was up-regulated after 6 hour of Al exposure in the root tip of 203B-14, which donates the favorable qALT5.03 allele. It is thus plausible to hypothesize that the induction of ZmNrat1 in the root tip of the 203B-14 could lead to Al tolerance based on a mechanism similar to that controlled by rice Nrat1, via Al transport reducing Al3+ concentration in the cell wall of apical cells22. Therefore, ZmNrat1 and ZmMATE3 could coordinate internal detoxification and exclusion mechanisms of Al tolerance, contributing to the high level of Al tolerance in 203B-14, conferred by qALT5.03.
The physical position of qALT8.05 (111.1–133.8 Mb) coincides with that of marker umc1202 at 109.2 Mb on chromosome 8, and umc1202 was linked with an Al tolerance QTL detected based on root re-growth and hematoxylin staining14. This suggests that qALT8.05 is conserved in different maize populations. In the 203B-14 × SCH3 population, qALT8.05 harbors GRMZM2G034421 that encodes a group III WRKY protein. ZmWRKY has 80% sequence identity with SbWRKY1, and 20.6% and 17.1% with OsWRKY22 and AtWRKY46, respectively. SbWRKY1 activates SbMATE expression in sorghum23, OsWRKY22 activates the expression of OsFRDL4 in rice27, whereas AtWRKY46 is a transcriptional repressor of AtALMT in Arabidopsis28, with all these genes being shown to control Al tolerance in the target species. Interestingly, ZmWRKY was induced by Al and showed higher expression in the Al-sensitive (SCH3) compared to the Al-tolerant parent. SCH3 donated the favorable allele of qALT8.05 that interacted with both qALT1.09 and qALT9.01 alleles, improving the RNRG in the population (Fig. 3a,b). These data suggest that ZmWRKY would be a candidate gene underlying qALT1.09, encoding an activator of Al tolerance genes located within qALT1.09 and qALT9.01 regions.
The Al tolerance QTL, qALT10.02 (8.7–13.5 Mb), was coincident with an Al tolerance QTL flanked by umc130 at 13.6 Mb, which was associated with an Al tolerance QTL in an F2 population derived from C100–6, another highly Al-tolerant Cateto line12. The predicted gene GRMZM2G068710, located within the qALT10.02 interval, encodes a C2H2 zinc finger protein with 59.4% of sequence identity to OsART1, a transcription factor that regulates several Al tolerance genes in rice24. ZmART1 was phylogenetically closer to OsART1 and clustered with ART1/STOP1 transcription factors from Arabidopsis (AtSTOP129), sorghum (SbSTOP130) and wheat (TaSTOP1A31), which have been shown to control Al tolerance in these species. Although controlling the expression of several genes under Al stress, OsART1 and AtSTOP1 were not responsive to Al in rice24 and Arabidopsis28, similarly to ZmART1 that was not differentially expressed by Al in both Kenyan maize lines. However, ZmART1 presented higher expression in 203B-14, which donated the favorable allele of qALT10.02. Additionally, qALT10.02 interacted with qALT1.09, with progeny double homozygous for the 203B-14 alleles at both QTL showing high Al tolerance. Thus, qALT10.02 allele from 203B-14 could enhance Al tolerance by harboring ZmART1, which would activate transcriptionally other genes.
A remarkable difference of this Kenyan population was the relative high effect of epistatic interactions between QTLs, which has never been detected in other mapping study of maize Al tolerance12,13,14,15. The existence of different Al tolerance mechanisms in Kenyan maize germplasm, independent from ZmMATE1, brings the opportunity to develop superior maize cultivars by introducing exotic lines harboring functional ZmMATE1 allele, such as Cateto Al237. Al-tolerant cultivars should benefit maize production on acidic soil regions worldwide.
Material and Methods
Plant material
The plant material consisted of 180 F2:3 progeny derived from a cross between Kenyan maize inbred lines previously characterized as extremely tolerant (203B-14) and sensitive (SCH3) to Al20. Additionally, the Brazilian standard lines for Al tolerance (Cateto Al237) and sensitivity (L53) were used as checks in hydroponics.
DNA extraction and markers genotyping
DNA was isolated from young leaves of the parental lines and F2 plants using a modified CTAB method as described by Saghai-Maroof et al.32. Genotyping of single nucleotide polymorphisms (SNPs) was performed using the Kompetitive Allele-Specific PCR (KASPTM) assays by LGC Genomics (www.lgcgenomics.com). The parental lines were screened with 1,250 random SNPs for polymorphism detection and F2 individuals were genotyped with 183 SNP markers.
Additionally, 14 fluorescently labeled SSR (Simple Sequence Repeat) markers were genotyped in the population. PCR reactions were performed using 50 ng of DNA, 1X PCR Buffer, 2.5 mM MgCl2, 166 µM of each dNTP, 0.2 μM of each primer and 0.5 U Taq DNA polymerase (Invitrogen, Thermo Fisher Scientific Inc.) in a final volume of 15 µL. Amplification cycles consisted of 95 °C for 2 minutes, eight cycles of 94 °C for 20 seconds, 60 °C (−1 °C/cycle) for 1 minute and 72 °C for 1 minute, followed by 35 cycles of 94 °C for 20 seconds, 53 °C for 1 minute and 72 °C for 1 minute, and a final extension of 72 °C for 5 minutes. PCR reactions were diluted in water (1:10), and 2 µL of each reaction were mixed with 0.3 µL of GeneTAB500 (Gene ID, São Carlos, Brazil) and 9.7 µL of 1% Tween 20. This mix was denatured at 94 °C for 5 minutes and loaded in a MegaBace 1000 DNA Analysis System (Amersham Biosciences, Thermo Fisher Scientific Inc.) with injection of 3 kV for 45 seconds and run at 10 kV for 75 minutes. The amplified fragments were analyzed using the software Fragment Profile 1.2 (Amersham Biosciences, Thermo Fisher Scientific Inc.). Sequence information and physical position of the SNPs and SSRs are available at Maize Genetics and Genomics Database (www.maizegdb.org/data_center/locus).
The PCR reaction to map ZmNrat1 was performed with 30 ng DNA, 1X PCR Buffer, 2 mM MgCl2, 125 µM of each dNTP; 0,5 µM of each primer F: 5′CGCGGAAACAGGAACCAAACCAAAA3′ and R: 5′CGGGTCTCTGCGTACCCCGA3′, 5% DMSO and 1U Taq DNA polymerase (Invitrogen, Thermo Fisher Scientific Inc.) in a final volume of 20 µL. Amplification cycles consisted of 95 °C for 2 minutes, 30 cycles of 94 °C for 30 seconds, 65 °C for 30 seconds and 72 °C for 1.5 minute, and a final extension of 72 °C for 5 minutes. The PCR product was cleaved with HinfI and the fragments were visualized in agarose gel 1.5% (w/v) stained with GelRed Nucleic Acid Stain (Biotium, Fremont, CA).
Evaluation of aluminum tolerance in nutrient solution
Al tolerance was assessed in a growth chamber under nutrient solution according to Guimaraes et al.15. Briefly, four-day old seedlings were transferred to polyethylene cups organized into containers filled with nutrient solution33 at pH 4.0 under continuous aeration. After 24 h of acclimatization, the initial root length (IRL) was measured and the seedlings were cultivated with and without {39} µM of Al3+ activity supplied as AlK(SO4)2.12H2O (brackets denote free Al3+ activity estimated with GEOCHEM-EZ software34 that corresponds to 222 µM of Al concentration). The final root length (FRL) of each seedling was measured five days after the treatments and net root growth (NRG) was calculated as FRL – IRL under Al treatment (NRG+Al) and control conditions, without Al (NRG−Al). The phenotypic index used to evaluate Al tolerance was Relative Net Root Growth (RNRG) calculated as NRG+Al/NRG−Al(x100).
The F2:3 progeny and the parents were evaluated in six experiments carried out in a completely randomized design with three replicates and two common checks (Cateto Al237 and L53). Analysis of variance was performed with RNRG data using PROC GLM of SAS software version 6.1.7601. Broad sense heritability (H2) was estimated based on family means.
Linkage analysis and QTL mapping
Marker loci were tested for goodness-of-fit to the expected single locus segregation ratio in an F2 population (1:2:1) using the chi-square test (P < 0.05). The linkage map was constructed using the MapMaker/EXP 3.035, with a minimum LOD of 3.0 and a maximum recombination frequency of 0.4. The Kosambi mapping function36 was used to convert recombination frequencies into map distances in centiMorgans (cM).
QTL mapping was performed using multiple interval mapping (MIM)37 implemented in QTL Cartographer version 2.5 for Windows38. The final model was selected using forward selection based on the Bayesian Information Criterion (BIC) with the penalty function c(n) = log(n), in which n = 180. The QTL position was defined based on the closest marker to the QTL maximum LOD value, and those markers were used to calculate the RNRG mean of genotypic classes for each QTL and for combinations of epistatic QTLs. Confidence intervals were established using the LOD-1 criterion39. The QTLs (q) were named using the acronym of Al tolerance (ALT) followed by their genetic position in chromosomal bins.
Searching for candidate genes within the Al tolerance QTLs
Genes previously associated with Al tolerance in other species were searched within the confidence intervals of Al tolerance QTLs based on sequence similarity using Phytozome (phytozome.jgi.doe.gov) considering the B73 genome sequence version 4.0.
Phylogenetic analysis of candidate genes
Protein sequences encoded by the candidate genes co-localized with Al tolerance QTLs and other similar sequences from maize were aligned with their respective homologs controlling Al tolerance in other plants using the M-COFFEE package available at T-COFFEE (tcoffee.crg.cat). Percent of identity was based on Clustal Omega (www.ebi.ac.uk/Tools/msa/clustalo/) and phylogenetic trees were constructed based on maximum likelihood using the software Mega v 10.0.540.
Expression analysis of candidate genes
The expression profiles of the candidate genes co-localized with Al tolerance QTLs were evaluated by quantitative real-time PCR (RT-qPCR) using the ABI Prism 7500 Fast System (Applied Biosystems, Thermo Fisher Scientific, Inc.). Maize seedlings were grown in hydroponics as described in the section Evaluation of aluminum tolerance in nutrient solution with seven seedlings representing each sample. The first centimeter of the root tips were collected after 0, 1, 6, 12 and 24 hours of treatment with and without {39} μM of Al3+ activity in the contrasting parents 203B-14 and SCH3. Total RNA was extracted using the RNeasy Plant Mini Kit (Qiagen, Germantown, MD) and the first-strand cDNA was synthesized using the High Capacity cDNA Reverse Transcription kit (Applied Biosystems, Thermo Fisher Scientific, Inc.) according to the manufacturer’s instructions. Transcripts were quantified using cDNA (5 ηg for target genes and 0.005 ηg for the endogenous control 18S rRNA), 2.5 μM of each primer and Fast SYBR Green Master Mix 1×(Applied Biosystems, Thermo Fisher Scientific, Inc.) in a final volume of 10 μL. Primers for each target gene were designed using the Primer-Blast tool (www.ncbi.nlm.nih.gov/tools/primer-blast/) (Supplementary Table S4). Calculation of relative gene expressions were performed using 2−ΔΔCt method41, with three biological and three technical replicates for each biological sample.
Data availability
The dataset for linkage and QTL mapping is included in this published article.
References
Awika, J. M. Major cereal grains production and use around the world. In Advances in Cereal Science: Implications to Food Processing and Health Promotion 1–13. American Chemical Society, https://doi.org/10.1021/bk-2011-1089.ch001 (ACS Publications, 2011).
Shiferaw, B., Prasanna, B. M., Hellin, J. & Bänziger, M. Crops that feed the world 6. Past successes and future challenges to the role played by maize in global food security. Food Security 3, 307 (2011).
Kanyanjua, S. M., Ireri, L., Wambua, S. & Nandwa, S. M. Acidic soils in Kenya: constraints and remedial options. KARI Technical Note 11 (2002).
Kisinyo, P. et al. Residual effects of lime and phosphorus application on soil and maize (Zea mays L.) performance in a Kenyan highlands acid soil. J. Agric. Pure Appl. Sci. Technol. 3, 1–10 (2009).
Borrero, J., Pandey, S., Ceballos, H., Magnavaca, R. & Bahia Filho, A. F. C. Genetic variances for tolerance to soil acidity in a tropical maize population. Maydica 40, 283–8 (1995).
von Uexküll, H. R. & Mutert, E. Global extent, development and economic impact of acid soils. Plant Soil 171, 1–15 (1995).
Kidd, P. S. & Proctor, J. Effects of aluminum on the growth and mineral composition of Betula pendula Roth. J. Exp. Botany 51, 1057–66 (2000).
Poschenrieder, C., Gunsé, B., Corrales, I. & Barceló, J. A glance into aluminum toxicity and resistance in plants. Sci. Total Environ. 400, 356–68 (2008).
Kochian, L. V., Piñeros, M. A., Liu, J. & Magalhaes, J. V. Plant Adaptation to acid soils: the molecular basis for crop aluminum resistance. Annu. Rev. Plant Biol. 66, 571–98 (2015).
Sousa, D. M. G., Miranda, L. N. & Oliveira, A. S. Acidez do solo e sua correção. In Fertilidade do Solo 205–274 (Sociedade Brasileira de Ciência do Solo, 2007).
Pandey, S. et al. Genetics of tolerance to soil fertility in tropical maize. Crop Sci. 34, 1511–4 (1994).
Sibov, S. T. et al. Two genes control aluminum tolerance in maize: genetic and molecular mapping analyses. Genome 42, 475–82 (1999).
Ninamango-Cárdenas, F. E. et al. Mapping QTLs for aluminum tolerance in maize. Euphytica 130, 223–32 (2003).
Conceição, L. D. H. C. S., Tessele, C. & Barbosa Neto, J. L. Diallel analysis and mapping of aluminum tolerance in corn inbred lines. Maydica 54, 51–64 (2009).
Guimaraes, C. T. et al. Genetic dissection of Al tolerance QTLs in the maize genome by high density SNP scan. BMC Genomics 15, 153 (2014).
Maron, L. G. et al. Two functionally distinct members of the MATE (multi-drug and toxic compound extrusion) family of transporters potentially underlie two major aluminum tolerance QTLs in maize. Plant J. 61, 728–40 (2010).
Zhang, X., Yan, L., Jingjing, H. & Jixing, X. Molecular mechanisms for coping with Al toxicity in plants. Int. J. Mol. Sci. 20, 1551 (2019).
Maron, L. G. et al. Aluminum tolerance in maize is associated with higher MATE1 gene copy number. Proc. Natl. Acad. Sci. USA 110, 5241–6 (2013).
Gudu, S., Maina, S. M., Onkware, A. O., Ombakho, G. & Ligeyo, D. O. Screening of Kenyan maize germplasm for tolerance to low pH and aluminium for use in acid soils of Kenya. Eastern Southern Africa Reg. Maize Conf. 17, 216–21 (2001).
Ouma, E. et al. Enhancing maize grain yield in acid soils of western Kenya using aluminium tolerant germplasm. J. Agric. Sci. Technol. 3, 33–46 (2013).
Matonyei, K. T. et al. The expression of ZmMATE1 gene at seminal root tip does not explain aluminum toxicity tolerance in a Kenyan maize breeding line. Inter. J. Sci. Res. Innovative Technol. 4, 45–59 (2017).
Xia, J., Yamaji, N., Kasai, T. & Ma, J. F. Plasma membrane-localized transporter for aluminum in rice. Proc. Natl. Acad. Sci. USA 107, 18381–5 (2010).
Melo, J. O. et al. Repeat variants for the SbMATE transporter protect sorghum roots from aluminum toxicity by transcriptional interplay in cis and trans. Proc. Natl. Acad. Sci. USA 116, 313–8 (2019).
Yamaji, N., Huang, C. F., Nagao, S., Yano, M. & Sato, Y. A zinc finger transcription factor ART1 regulates multiple genes implicated in aluminium tolerance in rice. Plant Cell 21, 3339–49 (2009).
Matonyei, K. T. et al. Physiological and molecular analysis of aluminum tolerance in selected Kenyan maize lines. Plant Soil 377, 357–67 (2014).
Xia, J., Yamaji, N., Che, J., Shen, R. F. & Ma, J. F. Differential expression of Nrat1 is responsible for Al-tolerance QTL on chromosome 2 in rice. J. Exp. Bot. 65, 4297–304 (2014).
Li, G. Z. et al. Transcription factor WRKY22 promotes aluminum tolerance via activation of OsFRDL4 expression and enhancement of citrate secretion in rice (Oryza sativa). New Phytol. 219, 149–62 (2018).
Ding, Z. J., Yan, J. Y., Xu, X. Y., Li, G. X. & Zheng, S. J. WRKY46 functions as a transcriptional repressor of ALMT1, regulating aluminum-induced malate secretion in Arabidopsis. Plant J. 76, 825–35 (2013).
Iuchi, S. et al. Zinc finger protein STOP1 is critical for proton tolerance in Arabidopsis and coregulates a key gene in aluminum tolerance. Proc. Natl. Acad. Sci. USA 104, 9900–5 (2007).
Huang, S., Gao, J. & You, J. Identification of STOP1-Like proteins associated with aluminum tolerance in sweet sorghum (Sorghum bicolor L.). Front. Plant. Sci. 9, 258 (2018).
Garcia-Oliveira, A. L. et al. Molecular characterization of TaSTOP1 homoeologues and their response to aluminium and proton (H+) toxicity in bread wheat (Triticum aestivum L.). BMC Plant Biol. 13, 134 (2013).
Saghai-Maroof, M. A., Soliman, K. M., Jorgenson, R. & Allard, R. W. Ribosomal DNA spacer length polymorphisms in barley: Mendelian inheritance, chromosomal location and population dynamics. Proc. Natl. Acad. Sci. USA 81, 8014–8 (1984).
Magnavaca, R., Gardner, C. & Clark, R. Evaluation of inbred maize lines for aluminum tolerance in nutrient solution. In Genetic Aspects of Plant Mineral Nutrition 255–65, https://doi.org/10.1007/978-94-009-3581-5_23 (Martinus Nijhoff, 1987).
Shaff, J. E. et al. GEOCHEM-EZ: a chemical speciation program with greater power and flexibility. Plant Soil 330, 207–14 (2010).
Lander, E. S. et al. Mapmaker: an interactive computer package for constructing primary genetic linkage maps of experimental and natural populations. Genomics 1, 174–81 (1987).
Kosambi, D. D. The estimation of map distances from recombination values. Ann. Eugenics 12, 172–5 (1944).
Kao, C. H., Zeng, Z. B. & Teasdale, R. D. Multiple interval mapping for quantitative trait loci. Genetics 132, 1203–16 (1999).
Wang, S., Basten, C. J. & Zeng, Z. B. Windows QTL Cartographer 2.5. Department of Statistics, North Carolina State University, Raleigh, NC. brcwebportal.cos.ncsu.edu/qtlcart/WQTLCart.htm (2012).
Lander, E. S. & Botstein, D. Mapping mendelian factors underlying quantitative traits using RFLP linkage maps. Genetics 121, 185–99 (1989).
Tamura, K., Stecher, G., Peterson, D., Filipski, A. & Kumar, S. MEGA6: Molecular Evolutionary Genetics. Analysis version 6.0. Mol. Biol. Evol. 30, 2725–9 (2013).
Livak, K. J. & Schmittgen, T. D. Analysis of relative gene expression data using real-time quantitative PCR and the 2−ΔΔCT method. Methods 25, 402–8 (2001).
Acknowledgements
The authors acknowledge financial support of Generation Challenge Programme, Fundação de Amparo à Pesquisa do Estado de Minas Gerais (Fapemig), Conselho Nacional de Desenvolvimento Cientifico e Tecnológico (CNPq), Coordenação de Aperfeiçoamento de Pessoal de Nível Superior (CAPES) and Embrapa.
Author information
Authors and Affiliations
Contributions
C.T.G., J.V.M., and S.O.G. conceived and designed experiments. T.K.M., B.A.B., R.G.N.G., E.O.O., R.K.C., L.C.A., D.O.L., B.A.W., P.O.K., A.O.O. and M.B.R.C. performed the experiments. T.K.M., B.A.B., R.G.N.G., R.W.N., and C.T.G. analyzed the data. T.K.M. and C.T.G. wrote the manuscript. S.O.G., B.A.B. and J.V.M. revised the manuscript. All authors read and approved the final manuscript.
Corresponding author
Ethics declarations
Competing interests
The authors declare no competing interests.
Additional information
Publisher’s note Springer Nature remains neutral with regard to jurisdictional claims in published maps and institutional affiliations.
Supplementary information
Rights and permissions
Open Access This article is licensed under a Creative Commons Attribution 4.0 International License, which permits use, sharing, adaptation, distribution and reproduction in any medium or format, as long as you give appropriate credit to the original author(s) and the source, provide a link to the Creative Commons license, and indicate if changes were made. The images or other third party material in this article are included in the article’s Creative Commons license, unless indicated otherwise in a credit line to the material. If material is not included in the article’s Creative Commons license and your intended use is not permitted by statutory regulation or exceeds the permitted use, you will need to obtain permission directly from the copyright holder. To view a copy of this license, visit http://creativecommons.org/licenses/by/4.0/.
About this article
Cite this article
Matonyei, T.K., Barros, B.A., Guimaraes, R.G.N. et al. Aluminum tolerance mechanisms in Kenyan maize germplasm are independent from the citrate transporter ZmMATE1. Sci Rep 10, 7320 (2020). https://doi.org/10.1038/s41598-020-64107-z
Received:
Accepted:
Published:
DOI: https://doi.org/10.1038/s41598-020-64107-z
- Springer Nature Limited