Abstract
Carboxylates are ideal directing groups because they are widely available, readily cleavable and excellent linchpins for diverse follow-up reactions. However, their use in meta-selective C−H functionalizations remains a substantial unmet catalytic challenge. Herein, we report the ruthenium-catalyzed meta-C–H alkylation of aromatic carboxylic acids with various functionalized alkyl halides. A bidentate N-ligand increases the electron density at the metal center of ortho-benzoate ruthenacycles to the extent that single-electron reductions of alkyl halides can take place. The subsequent addition of alkyl radicals is exclusively directed to the position para to the CAr–Ru bond, i.e., meta to the carboxylate group. The resulting catalytic meta-C−H alkylation extends to a wide range of (hetero)aromatic carboxylic acids including benzofused five-membered ring heteroarenes but no pyridine derivatives in combination with secondary/tertiary alkyl halides, including fluorinated derivatives. It also allows site-selective C5−H alkylation of 1-naphthoic acids. The products are shown to be synthetic hubs en route to meta-alkylated aryl ketones, nitriles, amides, esters and other functionalized products.
Similar content being viewed by others
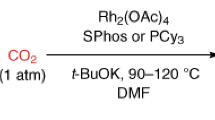
Introduction
The carboxylate group is a key functionality in many natural products, drugs and functional materials. Aromatic carboxylic acids are widely available in great structural diversity, are easily synthesized from readily available chemicals, can be interconverted into various other functional groups, and can be removed enabling their use as traceless directing group1. Due to these advantages, carboxylate groups are highly attractive directing groups for catalytic C–H activation reactions. However, the extension of catalytic concepts from strongly coordinating, often complex nitrogen donors to these simple, weakly coordinating functionalities is extremely challenging2,3. In 2007, the Daugulis and Yu group reported pioneering ortho-arylations of aromatic carboxylic acids in the presence of a palladium catalyst4,5. Since then, extensive research has led to the discovery of various catalytic ortho-C–H functionalization of native aromatic carboxylates in the presence of various metal catalysts2,3 (Fig. 1a). However, there are no transition-metal catalyzed processes, in which aromatic carboxylate groups direct C–H functionalizations into their meta-position.
Several strategies have been devised that direct catalytic C–H functionalizations of other aromatic compounds towards the position meta to a functional group6. Elegant proof-of-concept studies by the groups of Smith, Hartwig, and others based on steric or electronic control7,8,9,10,11, the group of Yu and others using template assistance12,13,14, the groups of Kuninobu, Kanai, and Phipps using non-covalent interactions15,16, and the groups of Yu and Dong using transient mediators17,18,19, along with Larrosa and other groups using tracelessly removable components20,21,22,23,24, have demonstrated that meta-C–H functionalization can be achieved. However, it has proved difficult to transfer these concepts from complex donor functionalities to native aromatic carboxylic acids.
A particularly attractive reaction concept has been realized by Ackermann25,26,27,28, Frost29,30,31, Liang32, Zhang33, and others34,35,36,37 based on ruthenium catalyst (Fig. 1b). In this σ-activation strategy, metal catalysts are directed towards the C–H group ortho to nitrogen/phosphine-based donor functionalities with formation of electron-rich ruthenacyles. These intermediates reduce electrophiles to form the corresponding radicals, which then attack the most reactive C–H group of the oxidized ruthenacycles I, i.e., the one in para-position to the CAr–RuIII bond. This allows the installation of alkyl groups meta to comparably compact ortho-directing groups (Fig. 1b). However, the strategy is so far limited to strongly coordinating, hard to install and remove functionalities such as pyridines26,27,28,29,30,31,32,33,34,35,36,37, imines25,32 and phosphines38,39. The use of desirable more ubiquitous functionalities such as carboxylates has not yet been achieved. It poses three substantial challenges: (1) As carboxylates are hard O-nucleophiles, they transform only slowly into metallacycles II22,40,41,42,43. This C–H activation step is rate-determining in most ortho-C–H functionalizations of carboxylates22,44. Instead, an esterification with the alkyl halide coupling partner is a common side reaction. (2) The ruthenacycles formed from aryl carboxylates are less electron-rich than those formed with nitrogen/phosphine-based direction groups. This lowers their redox potential and thus their capability to promote single-electron transfer to the electrophiles. Moreover, the position para to the Ru–C bond is less activated towards the addition of alkyl radicals in ortho-carboxylate ruthenacycles III than in electron-rich pyridine-bearing ruthenacycles (Fig. 1c).
We hypothesized that a catalytic concept for the so far elusive meta-C–H alkylation of aromatic carboxylate must address these issues in the following way: 1) The tendency of the alkyl halides to undergo an SN2 reaction with carboxylates must be reduced. 2) Electron-rich ligands must be added to increase the electron density of the resulting ruthenacycles, thus increasing the reduction potential of Int. II45 and the C–H reactivity of Int. II (Fig. 1c). 3) Non-covalent interactions between the carboxylate group and functional groups in the alkyl halides would be beneficial to bring the reactants into closer proximity46,47, thereby facilitating the single electron reduction and the radical addition steps. We herein report how this concept has led to the successful development of a meta-C–H alkylation of aromatic carboxylic acids with various 2o- and 3o-alkyl halides based on a ruthenium-catalyst activated by strongly coordinating, chelating bidentate N-ligand.
Results
Investigation of reaction conditions
We probed the feasibility of the envisioned reaction concept using the reaction of the amide-functionalized alkyl bromide 1a with benzoic acid 2a as the model. As expected, the state-of-the-art catalyst system that is highly efficient in pyridine-directed meta-C–H functionalizations ([Ru(p-cym)Cl2]2 as catalyst, K2CO3, Na2CO3 or KOAc as base, 1,4-dioxane as solvent) did not give any of the desired meta-C–H alkylation product 3aa26,27,29,30,33,34,35,36,37 (Table 1, entry 1, for details see Supporting Information, Supplementary Fig. 7). Instead, side reactions of the alkyl halide 1a, namely elimination (4a) with follow-up dimerization (5a) or dehalogenation (6a) were observed. We next probed whether the activity of the Ru-catalyst could be enhanced by the addition of coordinating ligands and found that bipyridines not only increased the overall conversion but also shifted the selectivity towards the desired product 3aa (entries 2-7). Best results were obtained with electron-rich bipyridine ligands L4 and L6, whereas electron-withdrawing substituents at the ligands lowered the catalytic efficiency (Table 1, entries 5-7). These results are consistent with our hypothesis that the electron density at the metal center of the ruthenacycle Int. II must be increased to facilitate the electron transfer to the electrophile. The solvent properties turned out to be the decisive factor for achieving the desired selectivity for a cross-coupling between 1a and 2a. A protic, relatively acidic reaction medium was found to be uniquely effective (entries 8-10). In a 9:1 tBuOH / HFIP solvent mixture, 3aa was formed almost exclusively, with the previously dominating side products 4a-6a being observed only in trace quantities. Reducing the pKa of the base led to a step-up in the yields, with best results being obtained with KOAc (entry 11). The presence of a potassium cation is crucial to achieve selectivity for the targeted cross-coupling. With other cations (Li, Zn, Mg), elimination 4a or dehalogenation products 6a were the predominant products (entries 12-14). This indicates that solvent-stabilized, potassium-bridged assemblies of the two substrates (II, III) are involved in the selectivity-determining steps. The high efficiency of a weak base and a proton-active solvent suggested that the release of product 3aa from III via protodemetalation and salt metathesis with 2a might still be sluggish. We, thus, added Lewis acids to facilitate these steps. Indeed, the presence of lithium bromide markedly improved the conversion without negatively affecting the selectivity (entries 15-18). This effect can be assigned to the Lewis acidic cation rather than the counter ion, because neither the addition of excess KBr nor the removal of bromide by silver salts has a decisive effect, whereas other Lewis acids such as Sc(OTf)3 were also beneficial (entries 17-19).
Substrate scope
With the optimized parameters established, we next explored the scope of the meta-alkylation with regard to the aromatic carboxylic acids (Fig. 2). Many ortho-substituted aromatic carboxylic acid were selectively converted into 1,2,3-trisubstituted arenes. The preference for these thermodynamically less favorable products indicates the sensitivity of the ortho-metalation step towards steric hindrance. Alkyl (3ba-ca), alkoxy (3da), fluoro (3ea) and chloro (3fa) substituents were all tolerated whereas-in analogy to related processes—nucleophilic hydroxyl and amino groups were found to be incompatible. The directed alkylation of meta-substituted aromatic carboxylic acids delivers 1,3,5-trisubstituted aromatic carboxylic acids (3ga−ia). Benzoates bearing para-substituents were also smoothly converted (3ja−pa), which shows that in contrast to the metalation, the radical addition step is not hampered by steric hindrance. Multi-substituted aromatic acids bearing various functional groups were also successfully converted into the corresponding products (3qa-va). The reaction extends to benzofused carboxylates and bicyclic heterocycles such as naphthalene (3wa), benzodioxane (3xa-za), 1-methylindole (3ab), benzofuran (3ac) and benzothiophene (3ad) but not yet to five- or six membered heteroarene carboxylates. Unfortunately, strongly coordinating 6-membered heterocycles such as pyridines or pyrimidines are not tolerated. The scalability of the protocol was demonstrated by a gram scale synthesis of 3ba (1.2 g, 68%).
[a]Reaction conditions: 1 (0.3 mmol), 2 (0.6 mmol), [Ru(p-cym)Cl2]2 (2.5 mol%), L6 (5.0 mol%), KOAc (2 equiv.), LiBr (30 mol%), tBuOH/HFIP = 9:1, 100 οC for 12 h under N2. Yields of the corresponding methyl esters after esterification with K2CO3 (2 equiv.) and MeI (5 equiv.) in NMP; [b]Isolated as the free acid; [c]1,4-dioxane instead of tBuOH/HFIP = 9:1; [d]1 (1.2 mmol), 2 (0.3 mmol), [Ru(p-cym)Cl2]2 (5.0 mol%), L5 (10.0 mol%), Na2CO3 (2 equiv.), AgOTf (20 mol%), tBuOH/HFIP = 20:1; [e][Ru(p-cym)Cl2]2 (5.0 mol%), L6 (10.0 mol%), AgOTf (20 mol%) instead of LiBr, tBuOH instead of tBuOH/HFIP.
We next investigated the scope of the reaction with regard to the alkyl bromide (Fig. 2). The presence of a coordinating functionality in the alkyl bromide was confirmed to be vital. Whereas t-butyl bromide gave no conversion, various tertiary α-bromo amides, esters, thioesters and ketones were smoothly transformed into the desired product (3ae-ax). Interestingly, even complex α-bromo amides derived from amino acids, such as Gly, Val, Asp, Ser, Phe and Met, all gave good yields (3ak−ap). Expectedly, competing esterification could not fully be suppressed for sterically less hindered secondary alkyl bromides (3ay), and esters were found as the main products for primary alkyl halides (3az). The selectivity for C–C coupling over esterification was found to be particularly high for difluoro alkyl electrophiles (3bb–mb), which substantially enhances the preparative utility of the transformation48. After all, α-CF2 carbonyl groups are desirable functionalities in drug discovery, as they are stable towards metabolic degradation via enolate mechanisms49,50. This moiety is found in the pharmacophores of several commercial drugs, e.g., Tafluprost and Gemcitabine. The meta-difluoroalkylation also extends to α-bromo fluorinated esters or amides derived from bioactive molecules such as L-menthol (3nb), galactolipin (3ob), borneol (3pb), estrone (3qb), mexiletine (3rb) and aminoglutethimide (3sb).
Another interesting observation was made when using 1-naphthoic acid as the substrate. In contrast to 2-napthoic acid, the carboxylate substituent directs the alkylation exclusively into the second aromatic ring, to the C5-position. The regioisomeric identity of the C5-alkylation product was unambiguously confirmed by single crystal X–ray diffraction analysis (Fig. 3). The C2 metalated intermediate IV, which should form preferentially, would deactivate the C5 position towards radical addition. Hence, one must assume that the cyclometallation is at least partially directed towards the C8 position. Intrigued by this unusual selectivity pattern, we extended the 1-naphthoic acid reaction variant to various α-bromo amides and esters (4aa−ua) including derivatives of borneol (4oa), L-menthol (4pa), galactolipin (4qa), mexiletine (4ra), estrone (4sa), cholesterol (4ta) and tocopherol (4ua).
[a]Reaction conditions: 1 (0.3 mmol), 2 (0.6 mmol), [Ru(p-cym)Cl2]2 (2.5 mol%), L5 (5.0 mol%), KOAc (2 equiv.), LiBr (30 mol%), tBuOH/HFIP = 9:1, 100 οC for 12 h under N2. Yields of the corresponding methyl esters after esterification with K2CO3 (2 equiv.) and MeI (5 equiv.) in NMP. [b]Isolated as the free acid. [c]1,4-dioxane instead of tBuOH/HFIP = 9:1.
Mechanistic investigations
As shown in Fig. 4, various control experiments were conducted to shed some light on the reaction mechanism: A) The attempted reaction of 2,6-dimethylbenzoic acid (2tb) or aromatic carboxylic acids with two meta-methyl substituents (2ub) gave no conversion, which confirms that the presence of ortho-C–H bonds is vital for the meta-functionalization to proceed. B) In the reaction of deuterated substrate 2a-[D5] with 1a, substantial D/H scrambling was observed in both ortho position at incomplete conversion. This indicates that the ortho C–H metalation step takes place rapidly and that it is reversible. C) A negligible kinetic isotope effect value (KIE) of 1.1 was observed when converting a mixture of 2a and 2a-[D5], which suggests that the reversible ortho-C − H bond insertion is fast and reversible. Without ligand, the H-D exchange of 2a-[D5] under standard conditions only gives 65% within 2 h. When L6 is added, the yield is increased to 95% (for details see Supporting Information), indicating that the bipyridine ligands accelerates the C–H activation step. D) When subjecting 1a along with a preformed cyclometallated carboxylate complex Ru-A to the reaction conditions, no conversion was observed. Only when ligand L6 was added, the product 3aa was formed in significant amounts. In combination with L6, Ru-A has a comparably high catalytic activity as [RuCl2(p-cym)]2. These findings support the intermediacy of ortho-benzoate ruthenacycles in the reaction and underline the vital importance of the bipyridine ligand L6 also for the steps following the ortho-metalation. In the reaction using [RuCl2(p-cym)]2, GC monitoring of the reaction revealed that p-cymene is liberated within the first minutes (for details see Supporting Information, Supplementary Fig. 11), indicating the displacement of this ligand with L6 during catalyst activation.
A No reaction without ortho C–H or two meta substituents. B H/D exchange experiment. C Kinetic isotopic effect study. D Stoichiometric and catalytic reaction of Ru-A. E Trapping of intermediate with 1,1-diphenylethylene. F The rate of product 5ab formation w/wo L6. G Cyclic voltammograms for 1a, [Ru(p-cym)Cl2]2 + w/wo L6 and Ru-A + w/wo L6. H Reversible H/D exchange. I Plausible catalytic cycle.
E) When conducting the reaction in the presence of the radical scavenger 1,1-diphenylethylene, meta-alkylation was retarded, and alkyl radical-capture product was detected. This finding supports the proposed radical mechanism. F) When the rate of the diphenylethylene adduct formation was monitored by in situ GC spectroscopy using a Ru-catalyst with and without ligand L6, it was found that ligand L6 accelerates this reaction. The same observations were made when employing the cyclometallated complex Ru-A as a catalyst (see the Supporting Information, Supplementary Table 10). G) Using cyclovoltammetry, the reduction potential of the alkyl halide 1a was determined as −1.01 V, and that of the [RuCl2(p-cym)]2 as −1.12 V. Thus, the [RuCl2(p-cym)]2 should already be able to reduce the alkyl halide to 1a. However, the addition of ligand L6 should facilitate this step, as it increases the reduction potential to −1.15 V. The preformed pyridine-stabilized, cyclometallated complex Ru-A has an even higher reduction potential of −1.22 V. H) When stirring 1-naphthoic acid 2am with D2O and the ruthenium catalyst, deuterium was incorporated only at the C2 in the absence of L5, whereas both C2 and C8 were fully deuterated in the presence of L5. This illustrates the crucial importance of the ligand for a successful functionalization in C5 position. I) Based on these control experiments, a plausible mechanism for meta-C−H alkylation of aromatic carboxylic acids is proposed. Initially, [Ru(p-cym)Cl2]2 reacts with L6 in the presence of KOAc to form the catalytically active monomeric species [Ru(L6)(OCOR)2]. The catalytically active complex [Ru(L6)(OCOR)2] first undergoes a reversible C − H activation to form ortho-carboxylate ruthenacycles II, in which the ligand increases the electron density of ruthenium center to facilitate the single-electron-reduction of 1a to afford alkyl radical and a ruthenacycles intermediate III. Then, the alkyl radical then attacks the para-position of the CAr–RuIII to form intermediate VI. In the next step, deprotonation with redox re-aromatization using KOAc produces intermediate VII, which undergoes protonation reactions to release the product 3aa and regenerate the catalyst [Ru(L6)(OCOR)2]. The Lewis acid LiBr helps to relocate the substrate, breaking the bridging coordination of the potassium and facilitating the release of product 3aa from VII via protodemetalation.
Applications
To illustrate the synthetic utility of the meta-C–H alkylated of aromatic carboxylic acid products, we performed various follow-up transformations of 3ba. As can be seen from Fig. 5, the carboxylate group can be reused as a directing group for ortho-C–H functionalization, such as arylations (6a)51, alkenylation/C–O cyclizations (6b)52, alkenylation/decarboxylations (6c)24, further increasing the complexity of the structures. The carboxylate group can also be removed tracelessly (6d)53 or serve as leaving group in a decarbonylative cyanation (6e)54. Furthermore, the carboxylate was reduced to the corresponding benzyl alcohol (6f)55 and to the aldehyde (6g)56. The carboxylate group was also transformed into a difluoromethyl ester (6h)57, a ketone (6i)58 and an amide (6j)59. These examples show the advantages of using such a versatile group as a carboxylic acid as a directing group in meta-C–H alkylations. In contrast to conventional directing groups, it opens up vast opportunities for follow-up reactions and can also be easily removed.
Discussion
A catalyst system generated from [RuCl2(p-cym)]2 and 5,5’-dimethylbipyridine was found to enable the meta-alkylation of aromatic carboxylic acids with secondary and tertiary alkyl bromides bearing various functional groups. Remarkably, 1-naphthoic acids direct the alkylation selectively into the C5-position, i.e, into the second aromatic ring. The reaction concept was shown to be broadly applicable to the synthesis of diversely functionalized arenes in substitution patterns that are not easily accessible otherwise. The carboxylate directing group is an ideal anchor point for follow-up functionalization, as demonstrated by the diversification of the products via various follow-up reaction. This makes the reaction ideal for late-state functionalizations of natural products or synthetic drugs.
Control experiments revealed that the reaction proceeds via rapid and reversible ortho-C–H metalation to give ortho-benzoate ruthenacycles, which reduce the alkyl halides to the corresponding radicals. These attack the ruthenacycles at the C–H bond para to the CAr–Ru bond allowing for a regiospecific synthesis of diversely functionalized C–H alkylated products from easily accessible starting materials. Adjusting the proton activity and basicity of the solvent system proved to be the decisive factor for achieving selectivity for C–H alkylation over intramolecular side reactions of the alkyl halides. Control experiments revealed the crucial role of potassium bases and the beneficial effect of Lewis acidic additives. They also revealed that bipyridine ligand accelerates the reversible cyclometallation step and facilitates the generation of the alkyl radicals by increasing the redox potential of the ruthenacycles.
Methods
Representative procedure for ruthenium catalyzed meta-C−H alkylation of (hetero)aromatic carboxylic acids
The procedure was conducted in a nitrogen-filled glove box. To a reaction vial equipped with a magnetic stir bar was added alkyl bromide 1 (0.3 mmol, 1.0 equiv.), aromatic carboxylic acids 2 (0.6 mmol, 2.0 equiv.), [Ru(p-cym)Cl2]2 (4.6 mg, 2.5 mol%), 5,5’-di-Me-bpy (2.8 mg, 5 mol%), KOAc (58.8 mg, 2.0 equiv.), LiBr (7.8 mg, 30 mol%), tBuOH (1.8 mL) and HFIP (0.2 mL). The reaction vial was sealed and removed from the glove box. The resulting mixture was stirred at 100 °C for 12 h, then quenched with HCl (1 M, 100 mL) and extracted with ethyl acetate (100 mL). The combined organic layers were dried over anhydrous MgSO4 and concentrated under reduced pressure.The residue was purified by flash column chromatography (petroleum ether/ethyl acetate/formic acid = 80/20/1) to give the corresponding products or the crude product was isolated as the corresponding methylation product to which K2CO3 (2 equiv.), MeI (5 equiv.) and NMP (2.0 mL) were added at 60 °C for a further 2 h. The mixture was then quenched with saturated sodium chloride solution (50 mL) and extracted with ethyl acetate (100 mL). The combined organic layers were dried over anhydrous MgSO4 and concentrated under reduced pressure. The residue was purified by column chromatography (petroleum ether/ethyl acetate) to give the alkylation product in the form of its methyl ester.
General procedure for ruthenium catalyzed C5-alkylation of 1-naphthoic acids
The procedure was conducted in a nitrogen-filled glove box. To a reaction vial equipped with a magnetic stir bar was added alkyl bromide 1 (0.3 mmol, 1.0 equiv.), aromatic carboxylic acids 2 (0.6 mmol, 2.0 equiv.), [Ru(p-cym)Cl2]2 (4.6 mg, 2.5 mol%), 4,4’-di-CF3-bpy (4.4 mg, 5 mol%), KOAc (58.8 mg, 2.0 equiv.), LiBr (7.8 mg, 30 mol%), tBuOH (1.8 mL) and HFIP (0.2 mL). The reaction vial was sealed and removed from the glove box. The resulting mixture was stirred at 100 °C for 12 h, then quenched with HCl (1 M, 100 mL) and extracted with ethyl acetate (100 mL). The combined organic layers were dried over anhydrous MgSO4 and concentrated under reduced pressure. The residue was purified by flash column chromatography (petroleum ether/ethyl acetate/formic acid = 80/20/1) to give the corresponding products or the crude product was isolated as the corresponding methylation product to which K2CO3 (2 equiv.), MeI (5 equiv.) and NMP (2.0 mL) were added at 60 °C for a further 2 h. The mixture was then quenched with saturated sodium chloride solution (50 mL) and extracted with ethyl acetate (100 mL). The combined organic layers were dried over anhydrous MgSO4 and concentrated under reduced pressure. The residue was purified by column chromatography (petroleum ether/ethyl acetate) to give the alkylation product in the form of its methyl ester.
Data availability
The authors declare that the data relating to the materials and methods, experimental procedures, NMR spectrum, mechanistic studies and synthetic application and X-ray structural analysis are available within this manuscript and in the Supplementary Information file. The X-ray crystallographic coordinates for structures 3ba, 3kb and 4aa reported in this study have been deposited at the Cambridge Crystallographic Data Centre (CCDC) under CCDC 2245724, CCDC 2284237 and CCDC 2245725, respectively. These data are available free of charge from the Cambridge Crystallographic Data Centre via www.ccdc.cam.ac.uk/data_request/cif. Data is also available from the authors upon request.
References
Gooßen, L. J. et al. New catalytic transformations of carboxylic acids. Pure. Appl. Chem. 80, 1725–1733 (2008).
Das, J., Mal, D. K., Maji, S. & Maiti, D. Recent advances in external-directing-group-free C–H functionalization of carboxylic acids without decarboxylation. Acs. Catal. 11, 4205–4229 (2021).
Pichette Drapeau, M. & Gooßen, L. J. Carboxylic acids as directing groups for C−H bond functionalization. Chem. Eur. J. 22, 18654–18677 (2016).
Giri, R. et al. Palladium-catalyzed methylation and arylation of sp2 and sp3 C−H bonds in simple carboxylic acids. J. Am. Chem. Soc. 129, 3510–3511 (2007).
Chiong, H. A., Pham, Q.-N. & Daugulis, O. Two methods for direct ortho-arylation of benzoic acids. J. Am. Chem. Soc. 129, 9879–9884 (2007).
Dutta, U., Maiti, S., Bhattacharya, T. & Maiti, D. Arene diversification through distal C(sp2)−H functionalization. Science 372, eabd5992 (2021).
Cho, J.-Y., Tse, M. K., Holmes, D., Maleczka, R. E. & Smith, M. R. Remarkably selective iridium catalysts for the elaboration of aromatic C–H bonds. Science 295, 305–308 (2002).
Ishiyama, T. et al. Mild iridium-catalyzed borylation of arenes. high turnover numbers, room temperature reactions, and isolation of a potential intermediate. J. Am. Chem. Soc. 124, 390–391 (2002).
Cheng, C. & Hartwig, J. F. Rhodium-catalyzed intermolecular C–H silylation of arenes with high steric regiocontrol. Science 343, 853–857 (2014).
Phipps, R. J. & Gaunt, M. J. A meta-selective copper-catalyzed C–H bond arylation. Science 323, 1593–1597 (2009).
Zhang, Y.-H., Shi, B.-F. & Yu, J.-Q. Pd(II)-catalyzed olefination of electron-deficient arenes using 2,6-dialkylpyridine ligands. J. Am. Chem. Soc. 131, 5072–5074 (2009).
Leow, D., Li, G., Mei, T.-S. & Yu, J.-Q. Activation of remote meta-C–H bonds assisted by an end-on template. Nature 486, 518–522 (2012).
Bera, M., Maji, A., Sahoo, S. K. & Maiti, D. Palladium(II)-catalyzed meta-C–H olefination: constructing multisubstituted arenes through homo-diolefination and sequential hetero-diolefination. Angew. Chem. Int. Ed. 54, 8515–8519 (2015).
Li, S., Cai, L., Ji, H., Yang, L. & Li, G. Pd(II)-catalysed meta-C–H functionalizations of benzoic acid derivatives. Nat. Commun. 7, 10443 (2016).
Kuninobu, Y., Ida, H., Nishi, M. & Kanai, M. A meta-selective C–H borylation directed by a secondary interaction between ligand and substrate. Nat. Chem. 7, 712–717 (2015).
Davis, H. J., Mihai, M. T. & Phipps, R. J. Ion pair-directed regiocontrol in transition-metal catalysis: A Meta-selective C–H borylation of aromatic quaternary ammonium salts. J. Am. Chem. Soc. 138, 12759–12762 (2016).
Wang, X.-C. et al. Ligand-enabled meta-C–H activation using a transient mediator. Nature 519, 334–338 (2015).
Dong, Z., Wang, J. & Dong, G. Simple amine-directed Meta-selective C–H arylation via Pd/norbornene catalysis. J. Am. Chem. Soc. 137, 5887–5890 (2015).
Li, G.-C., Wang, P., Farmer, M. E. & Yu, J.-Q. Ligand-enabled auxiliary-free meta-C−H arylation of phenylacetic acids. Angew. Chem. Int. Ed. 56, 6874–6877 (2017).
Cornella, J., Righi, M. & Larrosa, I. Carboxylic acids as traceless directing groups for formal meta-selective direct arylation. Angew. Chem. Int. Ed. 50, 9429–9432 (2011).
Luo, J., Preciado, S. & Larrosa, I. Overriding ortho-para selectivity via a traceless directing group relay strategy: the meta-selective arylation of phenols. J. Am. Chem. Soc. 136, 4109–4112 (2014).
Kumar, N. Y. P., Bechtoldt, A., Raghuvanshi, K. & Ackermann, L. Ruthenium(II)‐catalyzed decarboxylative C−H activation: versatile routes to meta‐alkenylated arenes. Angew. Chem. Int. Ed. 55, 6929–6932 (2016).
Zhang, J., Shrestha, R., Hartwig, J. F. & Zhao, P. A decarboxylative approach for regioselective hydroarylation of alkynes. Nat. Chem. 8, 1144–1151 (2016).
Huang, L., Biafora, A., Zhang, G., Bragoni, V. & Gooßen, L. J. Regioselective C−H hydroarylation of internal alkynes with arenecarboxylates: carboxylates as deciduous directing groups. Angew. Chem. Int. Ed. 55, 6933–6937 (2016).
Ackermann, L., Hofmann, N. & Vicente, R. Carboxylate-assisted ruthenium-catalyzed direct alkylations of ketimines. Org. Lett. 13, 1875–1877 (2011).
Li, J. et al. N-acyl amino acid ligands for ruthenium(II)-catalyzed meta-C–H tert-alkylation with removable auxiliaries. J. Am. Chem. Soc. 137, 13894–13901 (2015).
Ruan, Z. et al. Ruthenium(II)-catalyzed meta C−H mono- and difluoromethylations by phosphine/carboxylate cooperation. Angew. Chem. Int. Ed. 56, 2045–2049 (2017).
Ackermann, L., Korvorapun, K., Samanta, R. C. & Rogge, T. Remote C–H functionalizations by ruthenium catalysis. Synthesis 53, 2911–2946 (2021).
Saidi, O. et al. Ruthenium-catalyzed Meta sulfonation of 2-phenylpyridines. J. Am. Chem. Soc. 133, 19298–19301 (2011).
Paterson, A. J., St John-Campbell, S., Mahon, M. F., Press, N. J. & Frost, C. G. Catalytic meta-selective C–H functionalization to construct quaternary carbon centres. Chem. Commun. 51, 12807–12810 (2015).
Leitch, J. A. & Frost, C. G. Ruthenium-catalysed σ-activation for remote meta-selective C–H functionalisation. Chem. Soc. Rev. 46, 7145–7153 (2017).
Wang, X.-G. et al. Three-component ruthenium-catalyzed direct meta-selective C–H activation of arenes: a new approach to the alkylarylation of alkenes. J. Am. Chem. Soc. 141, 13914–13922 (2019).
Fan, Z., Ni, J. & Zhang, A. Meta-selective CAr–H nitration of arenes through a Ru3(CO)12-catalyzed Ortho-metalation strategy. J. Am. Chem. Soc. 138, 8470–8475 (2016).
Teskey, C. J., Lui, A. Y. W. & Greaney, M. F. Ruthenium‐catalyzed meta‐Selective C–H bromination. Angew. Chem. Int. Ed. 54, 11677–11680 (2015).
Yu, Q., Hu, L. A., Wang, Y., Zheng, S. & Huang, J. Directed meta-selective bromination of arenes with ruthenium catalysts. Angew. Chem. Int. Ed. 54, 15284–15288 (2015).
Li, G., Li, D., Zhang, J., Shi, D.-Q. & Zhao, Y. Ligand-enabled regioselectivity in the oxidative cross-coupling of arenes with toluenes and cycloalkanes using ruthenium catalysts: tuning the site-selectivity from the ortho to meta positions. ACS Catal. 7, 4138–4143 (2017).
Jing, K., Li, Z.-Y. & Wang, G.-W. Direct decarboxylative Meta-selective acylation of arenes via an Ortho-ruthenation strategy. Acs. Catal. 8, 11875–11881 (2018).
Li, G. et al. m-CAr–H bond alkylations and difluoromethylation of tertiary phosphines using a ruthenium catalyst. Org. Lett. 22, 9450–9455 (2020).
Fu, Y. et al. Remote C5-selective functionalization of naphthalene enabled by P–Ru–C bond-directed δ-activation. ACS Catal. 12, 5036–5047 (2022).
Cheng, G., Li, T.-J. & Yu, J.-Q. Practical Pd(II)-catalyzed C–H alkylation with epoxides: one-step syntheses of 3,4-dihydroisocoumarins. J. Am. Chem. Soc. 137, 10950–10953 (2015).
Zhang, Y.-H., Shi, B.-F. & Yu, J.-Q. Palladium(II)-catalyzed ortho-alkylation of benzoic acids with alkyl halides. Angew. Chem. Int. Ed. 48, 6097–6100 (2009).
Zhou, K. et al. Late-stage functionalization of aromatic acids with aliphatic aziridines: direct approach to form β-branched arylethylamine backbones. Acs. Catal. 9, 6738–6743 (2019).
Hu, X. Q., Hu, Z., Trita, A. S., Zhang, G. & Goossen, L. J. Carboxylate-directed C-H allylation with allyl alcohols or ethers. Chem. Sci. 9, 5289–5294 (2018).
Engle, K. M., Wang, D.-H. & Yu, J.-Q. Ligand-accelerated C−H activation reactions: evidence for a switch of mechanism. J. Am. Chem. Soc. 132, 14137–14151 (2010).
Dong, X.-Y., Li, Z.-L., Gu, Q.-S. & Liu, X.-Y. Ligand development for copper-catalyzed enantioconvergent radical cross-coupling of racemic alkyl halides. J. Am. Chem. Soc. 144, 17319–17329 (2022).
Hoque, M. E., Bisht, R., Haldar, C. & Chattopadhyay, B. Noncovalent interactions in Ir-catalyzed C–H activation: L-shaped ligand for para-selective borylation of aromatic esters. J. Am. Chem. Soc. 139, 7745–7748 (2017).
Bisht, R., Hoque, M. E. & Chattopadhyay, B. Amide effects in C-H activation: noncovalent interactions with L-shaped ligand for meta borylation of aromatic amides. Angew. Chem. Int. Ed. 57, 15762–15766 (2018).
Vaas, S. et al. Principles and applications of CF2X moieties as unconventional halogen bond donors in medicinal chemistry, chemical biology, and drug discovery. J. Med. Chem. 66, 10202–10225 (2023).
O’Hagan, D. Understanding organofluorine chemistry. An introduction to the C–F bond. Chem. Soc. Rev. 37, 308–319 (2008).
Gillis, E. P., Eastman, K. J., Hill, M. D., Donnelly, D. J. & Meanwell, N. A. Applications of fluorine in medicinal chemistry. J. Med. Chem. 58, 8315–8359 (2015).
Mei, R., Zhu, C. & Ackermann, L. Ruthenium(ii)-catalyzed C–H functionalizations on benzoic acids with aryl, alkenyl and alkynyl halides by weak-O-coordination. Chem. Commun. 52, 13171–13174 (2016).
Han, W. J., Pu, F., Fan, J., Liu, Z. W. & Shi, X. Y. Rhodium(III)‐catalyzed tandem C−H olefination and oxidative cyclization of aromatic acids with acrylates for the synthesis of (E)‐3‐ylidenephthalides. Adv. Syn. Catal. 359, 3520–3525 (2017).
He, Z. et al. A strategy for accessing aldehydes via palladium‐catalyzed C−O/C−N bond cleavage in the presence of hydrosilanes. Adv. Syn. Catal. 362, 5794–5800 (2020).
Xu, T. et al. Palladium-catalyzed decarbonylative cyanation of carboxylic acids with TMSCN. J. Org. Chem. 87, 11871–11879 (2022).
West, T. H. et al. Catalytic enantioselective [2,3]-rearrangements of allylic ammonium ylides: a mechanistic and computational study. J. Am. Chem. Soc. 139, 4366–4375 (2017).
Chen, D. et al. Triflylpyridinium enables rapid and scalable controlled reduction of carboxylic acids to aldehydes using pinacolborane. Angew. Chem. Int. Ed. 62, e202215168 (2022).
Krishnamurti, V., Barrett, C., Ispizua-Rodriguez, X., Coe, M. & Prakash, G. K. S. Aqueous base promoted O-difluoromethylation of carboxylic acids with TMSCF2Br: bench-top access to difluoromethyl esters. Org. Lett. 21, 9377–9380 (2019).
Wu, H. et al. One-pot synthesis of arylketones from aromatic acids via palladium-catalyzed suzuki coupling. J. Org. Chem. 81, 2987–2992 (2016).
Li, X. & Zou, G. Acylative Suzuki coupling of amides: acyl-nitrogen activation via synergy of independently modifiable activating groups. Chem. Commun. 51, 5089–5092 (2015).
Acknowledgements
The authors thank National Natural Science Foundation of China (No. 21971074, L.H.; 22001076, L.H.), Natural Science Foundation of Guangdong Province (No. 2022A1515010660, L.H.; 2021A1515220024, L.H.) and the Deutsche Forschungsgemeinschaft (DFG, German Research Foundation) under Germany’s Excellence Strategy−EXC 2033-390677874-RESOLV (L.-J.G.) for financial support.
Funding
Open Access funding enabled and organized by Projekt DEAL.
Author information
Authors and Affiliations
Contributions
X.L. and L.H. conceived and designed the experiments. X.L performed experiments and wrote the paper. Both L.H. and L.-J.G. revised reviewed and edited the paper. P.H., J.S., Y.K., and F.S. carried out the experiments. H.J. contributed to discussions. All authors discussed the results and commented on the manuscript. L.H. and L.-J.G. directed the whole project.
Corresponding authors
Ethics declarations
Competing interests
The authors declare no competing interests.
Peer review
Peer review information
Nature Communications thanks Zhong Jin, Worawat Niwetmarin and the other, anonymous, reviewer for their contribution to the peer review of this work. A peer review file is available.
Additional information
Publisher’s note Springer Nature remains neutral with regard to jurisdictional claims in published maps and institutional affiliations.
Supplementary information
Rights and permissions
Open Access This article is licensed under a Creative Commons Attribution 4.0 International License, which permits use, sharing, adaptation, distribution and reproduction in any medium or format, as long as you give appropriate credit to the original author(s) and the source, provide a link to the Creative Commons licence, and indicate if changes were made. The images or other third party material in this article are included in the article’s Creative Commons licence, unless indicated otherwise in a credit line to the material. If material is not included in the article’s Creative Commons licence and your intended use is not permitted by statutory regulation or exceeds the permitted use, you will need to obtain permission directly from the copyright holder. To view a copy of this licence, visit http://creativecommons.org/licenses/by/4.0/.
About this article
Cite this article
Luo, X., Hou, P., Shen, J. et al. Ligand-enabled ruthenium-catalyzed meta-C−H alkylation of (hetero)aromatic carboxylic acids. Nat Commun 15, 5552 (2024). https://doi.org/10.1038/s41467-024-49362-2
Received:
Accepted:
Published:
DOI: https://doi.org/10.1038/s41467-024-49362-2
- Springer Nature Limited