Abstract
Textured surfaces have been widely applied in many fields due to their excellent functional performances. Although several micro-scale surface texturing techniques have been used to fabricate surface textures, most are either very expensive, have material limitations, or lack flexibility. In this study, a novel textured surface generation method using vibration-assisted ball-end milling with a non-resonant vibrator is proposed. Firstly, the configuration of the vibration-assisted ball-end milling system is introduced. Then, the trajectories of the cutting edges are modeled and analyzed. Furthermore, an analysis of a non-resonant vibrator is conducted. Finally, surface texture machining experiments are conducted, and the feasibility of the proposed vibration-assisted ball-end milling method for surface texture fabrication is verified.
Highlights
-
A VA-BEM using a non-resonant vibrator was proposed for surface texture fabrication.
-
The tool trajectory in the VA-BEM process was modeled, and the quantitative relationships between the generated dimple geometric parameters and cutting and vibration parameters were built.
-
Experimental results were highly consistent with theoretical results, demonstrating that the proposed VA-BEM can precisely fabricate surface textures.
Similar content being viewed by others
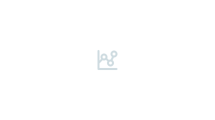
Avoid common mistakes on your manuscript.
1 Introduction
Textured surfaces can enhance the functionality and performance of industrial components and are widely used in aerospace, illumination, tribology, and biomedical and other engineering fields [1]. Bruzzone et al. [2] reported the main advances in engineered surface textures for functional performances. The improvement of tribological properties is a significant application of surface textures, which act as lubricant reservoirs and increase the film thickness between mating components to reduce surface contact [3, 4]. Ibatan et al. [3] reviewed commonly used surface texturing techniques in the last decades, including laser surface texturing [5,6,7], reactive ion etching [8], lithography, micro-casting [9], electrochemical machining [10], and micro-ball end milling [11,12,13,14]. These surface texturing techniques can be classified as non-mechanical and mechanical methods based on the material removal mechanism. Laser surface texturing as a non-mechanical method is a potential technology for fabricating textured surfaces on various materials, such as polymers, ceramics, and metals [15, 16]. Although laser ablation can improve machining efficiency and be applied to most materials, local microstructures and mechanical properties of the heat-affected zone may be changed due to material re-deposition caused by the high energy of laser surface texturing. Electrochemical processing of surface textures involves the removal of materials through anodic dissolution during electrolysis and is an easily controlled microfabrication technique [17]. This technique has several advantages compared to other surface texturing techniques benefiting from its high efficiency, low production cost, and absence of a heat-affected layer. However, this technique has some drawbacks, such as material limitations, electrode dissipation, and environmental pollution. Generally, the above-mentioned techniques are either unavailable for high-precision surface texture fabrication or only suitable for specific materials [18].
Recently, creating periodic dimples by micromechanical machining has been a promising approach. Matsumura et al. [19] presented a microdimple generation method on cylindrical surfaces using ball-end milling and established an analytical model to study the effects of cutting parameters on the topographies of the generated microdimples. Chen et al. [20] found that in the ball-end milling process, parameters such as tool radius, feed/pick ratio, and the initial cutting edge entrance angle affected the distribution of dimples. Kogusu et al. [13] pointed out that dimples can be generated on the metal surface using an inclined ball-end mill. They found that during cutter rotation, non-cutting time appears when the cutter tool is inclined and the depth of cut is less than the tool radius. Graham et al. [21] developed an efficient method to produce dimples with tilted micro-ball-end milling, and experimental results show that the friction coefficient of the dimple surfaces is lower than that of the corresponding flat surface. Arizmendi et al. [22] reported that a five-axis milling machine with a ball-end mill can effectively generate periodic elliptical dimples and developed a model to predict the geometry (shape, size, and orientation) of machined elliptical dimples by the five-axis milling on a flat surface. Although the ball-end milling method can be used to generate microdimples, the fabrication of textured surfaces requires a five-axis machine tool to adjust the ball-end milling cutter attitude to remove material, where the ball-end milling cutter position relative to the workpiece must be accurately controlled to achieve a uniform surface profile. However, machine tool characteristics, such as large inertia, backlash, friction, and stick slip, impose barriers to accurately and efficiently generate the desired micro-scale surface textures [18]. Additionally, high tool wear will affect the accuracy of the generated surface texture when machining hard-to-cut materials in the conventional milling process.
Vibration-assisted milling was proposed to overcome the above-mentioned problems, in which external vibrations with small amplitudes are applied to the milling process. The external vibrations are generated by vibrators, which can be classified into resonant and non-resonant types according to the working mode. The resonant vibrator works at its resonant frequency, whereas the non-resonant vibrator works at a non-resonant frequency. The vibrator is generally installed on the milling cutter or workpiece sides during the milling process. According to a previous study [23], this vibration-assisted milling method can improve the machining performance of hard-to-cut materials and can be used to generate surface textures. Ren et al. [24] studied the surface homogenization and morphology of a Ti-6Al-4 V alloy using longitudinal-torsional coupled ultrasonic vibration-assisted ball-end milling. They found that the mean cutting force decreased by ultrasonic vibration-assisted ball-end milling compared to the conventional milling process. Chen et al. [25] proposed an ultrasonic vibration-assisted milling method to fabricate surface textures on titanium alloy. Experimental results showed that an orderly scaly microtexture can be successfully generated by this method. Moreover, compared to the conventional milling process, ultrasonic vibration-assisted milling can effectively reduce the cutting force and the tool wear. Later, Zheng et al. [26] proposed an oblique rotary ultrasonic vibration-assisted milling method for the controllable fabrication of microstructures on the metallic surface. Experimental results showed that microstructures with much better geometric regularity can be obtained by eliminating secondary back-cut interference. These studies show that ultrasonic vibration-assisted milling is an effective method for surface texturing. However, these proposed methods encounter two critical issues that need to be solved. First, vibration is applied to the spindle of the machine tool; therefore, the spindle structure needs to be changed, which will increase the cost and affect the accuracy of the spindle. Second, the frequencies of these vibrators are fixed since they work in the vibrator’s resonant mode, which hinders their broad application in generating diverse and sophisticated surface textures. Furthermore, the resonant frequencies of these vibrators will be changed due to changes in different cutting tools and cutting parameters, which will affect the accuracies of machined surface textures. To solve the above-mentioned issues, non-resonant vibrators offer a good choice [27]. These work in the non-resonant mode and can deliver large vibration amplitudes while maintaining a high output stiffness to resist cutting forces during texturing. Another advantage of this vibrator is that it can use multiple frequencies simultaneously to generate arbitrary trajectories to fabricate sophisticated surface textures.
In this study, a vibration-assisted ball-end milling (VA-BEM) method is proposed for fabricating textured surfaces, in which a non-resonant vibrator is installed on the workpiece sides during the milling process. The organizational structure of this paper is as follows: the VA-BEM system is introduced in Sect. 2. In Sect. 3, a modal analysis of the non-resonant vibrator is conducted. Then, the amplitude calibration of the non-resonant vibrator is performed. Section 4 presents surface texture machining experiments. The conclusions of this work are drawn in Sect. 5.
2 Vibration-Assisted Ball-End Milling Method
To understand the surface texture generation process by the VA-BEM, the configuration of the VA-BEM system was introduced. Then, the trajectories of the cutting edges are modeled and analyzed.
2.1 Configuration of the VA-BEM System
To build the VA-BEM system, a non-resonant vibrator was used in this study, as shown in Fig. 1, which was designed in previous works [18, 28]. In previous studies, this non-resonant vibrator was used for turning-based surface texturing, whereas the feasibility of using this non-resonant vibrator for milling-based surface texture fabrication will be explored in this study. Compared to the turning-based surface texturing process, milling-based surface texturing has a higher cutting speed, which ensures higher machining efficiency and surface finish. As shown in Fig. 1, the non-resonant vibrator consists of two symmetrical compound bridge (CB) mechanisms driven by two piezoelectric actuators (PEAs) and a Z-shaped flexure hinge (ZFH) mechanism. A three-axis milling machine tool with a ball-end milling cutter installed on the spindle was used during surface texturing. The non-resonant vibrator was fixed on the moving workbench of the machine tool, while the workpiece was set on the non-resonant vibrator (Fig. 1). The workpiece was installed in the middle of the ZFH mechanism. When two identical voltages are applied to the PEAs, two CB mechanisms oscillate at the same phase. The outputs of two symmetric CB mechanisms are applied to the two input ends of the ZFH mechanism; the flexure hinge will bend and generate displacement at the workpiece in the Z-direction. Therefore, by controlling the applied voltages of two PEAs, the workpiece can realize vibration toward the direction of depth of cut with different amplitudes.
2.2 Modeling of the Tool Trajectory
Based on the configuration of the VA-BEM system, the coordinate systems of VA-BEM are defined as shown in Fig. 2. To describe the tool motion, both the tool coordinate system (\(O_{T} - X_{T} Y_{T} Z_{T}\)) and the workpiece coordinate system (\(O_{W} - X_{W} Y_{W} Z_{W}\)) are defined as shown in Fig. 2a. The tool coordinate is defined with its origin,\(O_{T}\), located at the cutting edge fillet center and its \(Z_{T}\) axis set in the reverse depth-of-cut direction. The \(X_{T}\) axis has a radial direction and is tangent to the projection of one cutting edge. The \(Y_{T}\) axis is perpendicular to the \(X_{T}\) and \(Z_{T}\) axes according to the right-hand rule. The workpiece coordinate system (\(O_{W} - X_{W} Y_{W} Z_{W}\)) is set up with its origin located at the right face of the workpiece and with its \(Z_{W}\) axis in the reverse depth-of-cut direction. The \(Y_{W}\) axis coincides with the feed direction of the cutting tool, and the \(X_{W}\) axis is determined according to the right-hand rule. A schematic three-dimensional (3D) view of a ball-end mill with a nominal radius \(R\) and helix angle \(i_{0}\) is shown in Fig. 2b. For clarity, only one cutting edge is plotted in the figure, but the developed model is generalized to an \(N_{t}\)-tooth ball-end mill. It is assumed that the cutting edge shown in Fig. 2b is the cutting edge \(k\), where \(k\) values can be 1, 2, …, \(N_{t}\) [22]. A front view of the radial plane at the angular position \(\beta_{i}\) is shown in Fig. 2c. To define the position of any point located on the cutting edge \(k\), a local coordinate system \(O_{k} - X_{k} Y_{k} Z_{k}\) established on the cutting edge \(k\) needs to be determined. The local coordinate system is constructed with the cutting edge fillet center \(O_{T}\) as the origin. The \(Z_{k}\)- axis coincides with the tool axis \(Z_{T}\). The \(X_{k}\)-axis has a radial direction and is tangent at point \(O_{k}\) to the projection of the cutting edge \(k\) into the plane perpendicular to the \(Z_{k}\)- axis. The \(Y_{k}\)- axis is determined based on the right-hand rule.
In the local coordinate system \(O_{k} - X_{k} Y_{k} Z_{k}\), the cutting edge \(k\) is divided into a finite number of points \(P_{i}\), where \(i = 1,2,...,n,\) and n is the number of points into which the cutting edge is divided. The position of a cutting edge point \(P_{i}\) is determined by the height \(z_{i}\), radial position \(R_{i}\), and angular position \(\beta_{i}\). The height \(z_{i}\) of point \(P_{i}\) and radial position \(R_{i}\) can be defined by the following equations:
Any cutting edge point \(P_{i}\) in the local coordinate system \(O_{k} - X_{k} Y_{k} Z_{k}\) can be described by the following equations.
Based on the transformation relationship between the local coordinate system and the tool coordinate system, the coordinates of the cutting edge point \(P_{i}\) in the tool coordinate system \(O_{T} - X_{T} Y_{T} Z_{T}\) can be described by the following equations.
where \(\varphi\) is the angle between the adjacent cutting edges. For the cutting tool with two cutting edges, it can be expressed as follows:
In the VA-BEM process, the ball-end milling cutter moves along the \(Y_{W}\) axis, and the workpiece vibrates longitudinally along the \(Z_{W}\) axis. Based on the transformation relationship between the tool coordinate system and the workpiece coordinate system, the coordinates of any cutting edge point \(P_{i}\) can be obtained using the following coordinate transformation equations:
where \({}_{T}^{Z} {\varvec{R}}(\alpha ),{}_{T}^{Y} {\varvec{R}}(\theta ),{}_{T}^{X} {\varvec{R}}(\gamma )\) are the coordinate transformation matrix induced by tool rotation and tool axis inclination, \(f\) is the frequency (Hz), \(f_{r}\) is the feed rate in the feed direction (μm/s), \(A\) is the vibration amplitude of the workpiece and \(t_{i}\) is time (s), \(n\) is the speed of the spindle (rpm) and \(\omega\) is the angular velocity of the spindle (rad/s). \([\begin{array}{*{20}c} {x_{0} } & {y_{0} } & {z_{0} } \\ \end{array} ]\) is the initial position of the cutting edge in the workpiece coordinate system \(O_{W} - X_{W} Y_{W} Z_{W}\). \({}_{T}^{Z} {\varvec{R}}(\alpha ),{}_{T}^{Y} {\varvec{R}}(\theta ),{}_{T}^{X} {\varvec{R}}(\gamma )\) are expressed as follows:
where \(\alpha\) is the rotation angle of the ball-end milling cutter (rad), \(\theta\) is the tilt angle (rad), and \(\gamma\) is the yaw angle (rad). Since the ball-end milling cutter is installed along the spindle,\(\theta = 0,\gamma = 0\) in this study. \(\alpha\) is expressed as follows
To analyze the effect of the vibration on the generated trajectory, two points on the cutting edge are selected, and the generated trajectories are calculated based on Eq. (6), as shown in Fig. 3. A top view of trajectories in the XWYW plane is shown in Fig. 3a. Since the vibration is added in the depth of cut direction, the vibration does not affect the tool trajectory in this XWYW plane. The velocity of the ball-end milling cutter relative to the workpiece in the feed direction of the VA-BEM process is a constant value. Thus, the trochoidal trajectory of the cutting edge point is generated, as shown in Fig. 3a, which shows the same trajectory of cutting edge points as in conventional milling. To analyze the effect of the vibration on the generated trajectory of the cutting edge in the YWZW plane, the generated trajectories are shown in Fig. 3b. Periodic variations in trajectory due to vibration are observed in the ZW-axis direction; therefore, there is a relative motion in the depth of cut direction between the ball-end milling cutter and the workpiece. Thus, periodic surface textures can be generated benefiting from this periodic variation of trajectories in the depth of cut direction.
To further analyze the effects of the cutting and vibration parameters on the topography of surface textures, the cutting tool sweep trajectories have been studied. In this study, a cutting tool with two cutting edges was investigated. In the workpiece coordinate system, coordinates of cutting edges at a particular time can be calculated based on Eq. (6) and displayed in Fig. 4a, b in different views. Then, the sweep trajectory of the cutting tool can be generated (shown in Fig. 4c) by considering all coordinates of the cutting edge with time. From Fig. 4c, it can be seen that the bottom of the cutting edge sweep trajectory forms a periodic valley shape, which will remove the corresponding workpiece material to generate periodic surface textures.
Furthermore, the quantitative relationships between the generated dimple geometric parameters (length l and depth \(D\)) and cutting and vibration parameters have been built, which are expressed as:
where Ap is the depth of cut. From Eq. (11), it can be found that the generated microdimples can be controlled by cutting and vibration parameters. The length l of the microdimple is related to the depth \(D\) of the microdimple and the diameter of the cutting tool R. The depth \(D\) of the microdimple is determined by the vibration amplitude of the workpiece and the depth of cut. Hence, the distribution and shape of microdimples can be controlled by carefully selecting cutting and vibration parameters.
3 Analysis of the Non-resonant Vibrator
3.1 Modal Analysis of the Non-resonant Vibrator
To ensure the stability of the non-resonant vibrator during the surface texturing process, the operating frequency of the non-resonant vibrator should be controlled under its first resonant frequency. After the workpiece is fixed on the non-resonant vibrator, the first resonant frequency of the non-resonant vibrator will be changed. Therefore, modal analysis has been conducted with a workpiece fixed on the output of the non-resonant vibrator. The modal analysis of the non-resonant vibrator was carried out using ANSYS Workbench software. During the modal analysis, the materials were set as spring steel, and the parameters were set as follows: density was 7800 kg/m3, Young’s modulus was 2.0 × 1011 Pa, Poisson’s ratio was 0.3, and yield strength was 784 MPa. Then, the mesh of the model was generated. Since the non-resonant vibrator was fixed to the three-axis machine tool using screws through threaded holes on the two CB mechanisms, fixed constraints were applied to the four threaded holes during the simulation process.
The modal analysis results, including the resonant frequencies and modal shapes, are shown in Fig. 5. The first resonant frequencies in the depth of cut and feed directions are 651.19 and 537.08 Hz, respectively. Therefore, during the microdimple marching process, the vibration frequency of the non-resonant vibrator should be controlled at less than 537.08 Hz to keep the stability of the non-resonant vibrator.
3.2 Displacement Calibration of the Non-resonant Vibrator
To control the surface topography of generated surface textures, it is necessary to determine the vibration trajectory of the workpiece by calibrating the amplitude of the non-resonant vibrator. The experimental setup is shown in Fig. 6. The displacement calibration is conducted in the workpiece coordinate system (\(O_{W} - X_{W} Y_{W} Z_{W}\)), as shown in the figure. Vibration trajectories were measured using two Micro-Sense capacitance sensors (Model: 5504, USA), which are arranged along \(Y_{W}\) and \(Z_{W}\) axis directions, respectively. The measuring range of the capacitance sensors is 100 μm, with 100-kHz bandwidth and sub-nanometer resolution.
Two sinusoidal signals with a phase difference of 0 deg were generated in LabVIEW software and sent to a data acquisition card (NI PCIe-6361, USA). The signal was amplified through a piezo-amplifier (Trek PZD350A, Advanced Energy, Denver, CO, USA) to excite piezoelectric actuators. Simultaneously, the vibration displacements were collected at a sampling frequency of 10 kHz using the same data acquisition card. Four cases with the applied sinusoidal voltages of 100, 200, 300, and 400 V (peek-to-valley) were studied, and corresponding displacements in the depth of cut direction were collected. The vibration displacement result of the non-resonant vibrator with 400-V voltage is illustrated as one case of amplitude calibration, as shown in Fig. 7a. It is demonstrated that the vibration displacement of the non-resonant vibrator changes with time as a sinusoidal shape. The peak-to-valley displacement values for all cases are extracted and depicted in Fig. 7b. It can be seen that the peak-to-valley displacement of the non-resonant vibrator linearly increases with increasing input voltage. Then, the peak-to-valley displacement values of experiments are linearly fitted as a function of input voltage, which can be expressed as:
where \(y\) denotes the peak-to-valley displacement values (μm) of the non-resonant vibrator and \(x\) represents the input voltage of PEAs.
In the depth-of-cut direction, the vibration amplitude increases with increasing voltage. Thus, different vibration amplitudes can be obtained by controlling the input voltage amplitude.
4 Surface Texture Machining Experiments
4.1 Experimental Setup
Surface texture machining experiments were conducted at a HAAS CNC milling machining center. The experimental setup is shown in Fig. 8. The non-resonant vibrator was fixed on the moving workbench of the machine tool. The workpiece was set on the non-resonant vibrator, and the material was chosen as Aluminum 6061. The diameter of the ball-end milling cutter was selected as 3 mm. Before the surface texturing experiments, a pre-machining was conducted to remove the effects caused by the mounting and workpiece geometrical errors.
Surface texturing experiments were performed on the flat surface of the workpiece. Based on Eq. (11), the depth of cut directly affects the length and depth of machined microdimples. Therefore, different depths of cut were used to study its effects on the generated dimples. The cutting and vibration parameters are listed in Table 1. For cases 1 to 4, the input voltages were all set as constant as 300 V (peak-to-valley), and the corresponding peek-to-valley displacement was 21.54 μm based on the displacement calibration results in Sect. 3.2. The depth of the cut was set as − 20.32, − 10.16, 0, and 10.16 μm, respectively. The experimental vibration frequencies of the cases were all set as constant at 1 Hz by controlling the frequency of the output voltage of the piezo-amplifier (Trek PZD350A, Denver, Co, USA).
4.2 Results and Discussion
After the machining experiments, the workpieces were cleaned in an ultrasonic washing machine and measured using a white light interferometer (Zygo New view 7300). The measured results from cases 1 to 4 are shown in Fig. 9. As shown in Fig. 9a, when the depth of cut, Ap, is 10.16 μm, microdimples are not connected and are discretely distributed on the surface of the workpiece. According to a previous study [21], the friction coefficient of dimple surfaces is lower than that of the corresponding smooth surfaces. Recently, there has been a growing tendency to design more intricate hierarchical surfaces to satisfy new and multiple functionalities. They are usually in the form of multiple dimples merged to form a blended texture in a regular pattern, allowing multiple functionalities [18]. With the increasing of depth of cut, Ap, microdimples gradually approach and overlap, and a blended texture of channels and dimples can be generated in one step. Such textured surfaces may find potential applications in microfluidic transport [29] and manipulated optical properties in optics [30]. Additionally, the hierarchical nature of the texture, similar to “valley-like” formations, serves as air pockets that resist contact between the liquid and the surface, potentially enhancing hydrophobicity [31]. Additionally, there is no burr observed for all cases, which means this VA-BEM can fabricate surface texture with good surface quality.
To quantify the geometrical parameter of generated surface textures, the cross-sectional profiles of surface textures are extracted for all cases and depicted in Fig. 10. Based on the extracted cross-sectional profiles, the depth (D) and length (l) of dimples can be measured. To eliminate the measurement errors, three dimples for each case were measured. The measured results are listed in Table 2. The average values of the measured dimple depths from cases 1 to 4 are 42.29, 32.27, 21.87, and 11.58 μm, respectively, while the corresponding standard deviations are 1.14, 0.75, 0.93, and 0.45, respectively. The average values of measured dimple length from cases 1 to 4 are 706, 614.67, 505.67, and 366 μm, respectively, while the corresponding standard deviations are 6.55, 5.50, 3.78, and 3.6, respectively.
Based on the measured results in Table 2 and the corresponding theoretical results according to Eq. (11), histograms of the geometrical parameters of the generated dimples are displayed in Fig. 11. For the depth of dimples of cases 1–4, the relative errors between the measured average experimental results and corresponding theoretical results are 1.04%, 1.8%, 1.53%, and 1.76%, respectively. For the length of dimples of cases 1–4, relative errors between the measured average experimental results and corresponding theoretical results are 0.2%, 0.2%, 0.1%, and 0.7%, respectively. Therefore, experimental results are highly consistent with theoretical results, demonstrating that the proposed VA-BEM can precisely fabricate surface textures.
5 Conclusions
In this study, a VA-BEM using a non-resonant vibrator was proposed for surface texture fabrication. The configuration of the VA-BEM system was first introduced and then the tool trajectory in the VA-BEM process was modeled. Based on the tool trajectory model, the effect of the vibration on the tool trajectory was analyzed. Then, the sweep trajectory of the cutting tool was generated by considering all coordinates of the cutting edge with time. The envelope of the cutting edge at the bottom of the sweep trajectory formed a periodic valley shape, which can be used to remove the corresponding workpiece material to generate periodic surface textures, illustrating the working principle of the VA-BEM. Furthermore, the quantitative relationships between the generated dimple geometric parameters and cutting and vibration parameters were built, demonstrating that the generated microdimples can be controlled by cutting and vibration parameters. Modal analysis was conducted with a workpiece fixed on the output of the non-resonant vibrator. The modal analysis results showed that the non-resonant vibrator should be controlled at less than 537.08 Hz to keep the stability of the non-resonant vibrator. Finally, surface texturing experiments were conducted to validate the feasibility of the proposed VA-BEM for surface texture generation. Experimental results showed that for the depth of dimple, relative errors between the measured average experimental result and corresponding theoretical results were less than 1.8%, while for the length of dimples, relative errors between the measured average experimental results and corresponding theoretical results were less than 0.7%. Experimental results were highly consistent with theoretical results, demonstrating that the proposed VA-BEM can precisely fabricate surface textures. Such textured surfaces may find potential applications in tribology, microfluidic transport, and hydrophobicity fields.
Availability of data and materials
The authors declare that all data supporting the findings of this study are available within the article.
References
Yuan Y, Zhang D, Jing X et al (2019) Fabrication of hierarchical freeform surfaces by 2D compliant vibration-assisted cutting. Int J Mech Sci 152:454–464
Bruzzone AAG, Costa HL, Lonardo PM et al (2008) Advances in engineered surfaces for functional performance. CIRP Ann 57(2):750–769
Ibatan T, Uddin MS, Chowdhury MAK (2015) Recent development on surface texturing in enhancing tribological performance of bearing sliders. Surf Coat Technol 272:102–120
Hassan S, Ghani J, Che Haron CH et al (2017) A review of the milling process to fabricate a dimple structure. J Mech Eng 1:175–192
Wakuda M, Yamauchi Y, Kanzaki S et al (2003) Effect of surface texturing on friction reduction between ceramic and steel materials under lubricated sliding contact. Wear 254(3–4):356–363
Podgornik B, Sedlacek M (2012) Performance, characterization and design of textured surfaces. J Tribol 134:041701
Rapoport L, Moshkovich A, Perfilyev V et al (2008) Friction and wear of MoS2 films on laser textured steel surfaces. Surf Coat Technol 202(14):3332–3340
Wang X, Kato K, Adachi K (2002) The lubrication effect of micro-pits on parallel sliding faces of SiC in water. Tribol Trans 45(3):294–301
Cannon AH, King WP (2009) Casting metal microstructures from a flexible and reusable mold. J Micromech Microeng 19(9):095016
Yan D, Qu N, Li H et al (2010) Significance of dimple parameters on the friction of sliding surfaces investigated by orthogonal experiments. Tribol Trans 53(5):703–712
Yan J, Horikoshi A, Kuriyagawa T et al (2012) Manufacturing structured surface by combining microindentation and ultraprecision cutting. CIRP J Manuf Sci Technol 5(1):41–47
Wang Y, Zhou TF, Zhou J et al (2022) Precision milling of integrated turbine based on a non-contact on-machine measurement system. Nanomanuf Metrol 5(4):394–402
Kogusu S, Ishimatsu T, Ougiya Y et al (2008) Decoration of metal surface by dimples using ball-end milling process. In: 2008 IEEE international conference on industrial technology. IEEE, pp 1–6
Wang W, Huang Z, Shen D et al (2013) The effect of triangle-shaped surface textures on the performance of the lubricated point-contacts. J Tribol 135:2
Voevodin AA, Zabinski JS (2006) Laser surface texturing for adaptive solid lubrication. Wear 261(11–12):1285–1292
Avanish KD, Vinod Y (2008) Laser beam machining—a review. Int J Mach Tools Manuf 48(6):609–628
Qu N, Chen X, Li H et al (2014) Electrochemical micromachining of micro-dimple arrays on cylindrical inner surfaces using a dry-film photoresist. Chin J Aeronaut 27(4):1030–1036
Yuan Y, Zhang D, Jing X et al (2019) Micro texture fabrication by a non-resonant vibration generator. J Manuf Process 45:732–745
Matsumura T, Takahashi S (2012) Micro dimple milling on cylinder surfaces. J Manuf Process 14(2):135–140
Chen JS, Huang YK, Chen MS (2005) A study of the surface scallop generating mechanism in the ball-end milling process. Int J Mach Tools Manuf 45(9):1077–1084
Graham E, Park CI, Park SS (2013) Fabrication of micro-dimpled surfaces through micro ball end milling. Int J Precis Eng Manuf 14(9):1637–1646
Arizmendi M, Jiménez A, Cumbicus WE et al (2019) Modelling of elliptical dimples generated by five-axis milling for surface texturing. Int J Mach Tools Manuf 137:79–95
Chen W, Huo D, Shi Y, Hale JM (2018) State-of-the-art review on vibration-assisted milling: principle, system design, and application. Int J Adv Manuf Technol 97:2033–2049
Ren W, Xu J, Lin J et al (2019) Research on homogenization and surface morphology of Ti-6Al-4V alloy by longitudinal-torsional coupled ultrasonic vibration ball-end milling. Int J Adv Manuf Technol 104(1):301–313
Chen P, Tong J, Zhao J et al (2020) A study of the surface microstructure and tool wear of titanium alloys after ultrasonic longitudinal-torsional milling. J Manuf Process 53:1–11
Zheng Z, Zhang J, Feng P et al (2023) Controllable fabrication of microstructures on the metallic surface using oblique rotary ultrasonic milling. Int J Mech Sci 237:107805
He Y, Zou P, Zhu Z et al (2018) Design and application of a flexure-based oscillation mechanism for surface texturing. J Manuf Process 32:298–306
Zhu WL, Zhu Z, He Y et al (2017) Development of a novel 2-D vibration-assisted compliant cutting system for surface texturing. IEEE ASME Trans Mechatron 22(4):1796–1806
Denkena B, Kästner J, Wang B (2010) Advanced microstructures and its production through cutting and grinding. CIRP Ann 59(1):67–72
Malshe A, Rajurkar K, Samant A et al (2013) Bio-inspired functional surfaces for advanced applications. CIRP Ann 62(2):607–628
Ng MK, Saxena I, Ehmann KF et al (2016) Improving surface hydrophobicity by microrolling-based texturing. J Micro Nano Manuf 4:3
Acknowledgements
The authors gratefully acknowledge supports of the National Natural Science Foundation of China (Grant No. 52105452), High Level Personnel Project of Jiangsu Province (Grant No. JSSCBS20210188). Central University Basic Research Fund of China (Grant No. NT2022017), Jiangsu Key Laboratory of Precision and Micro-Manufacturing Technology (Grant No. 56XAC22006), Nanjing Foundation for Returnees (Grant No.1005-YQR21043), Foundation of Jiangsu Science and Technology Association Young Talents ((Grant No. JSTJ-2022-073).
Author information
Authors and Affiliations
Contributions
All authors read and approved the final manuscript.
Corresponding author
Ethics declarations
Competing interest
The authors declare that they have no competing interest.
Additional information
Publisher's Note
Springer Nature remains neutral with regard to jurisdictional claims in published maps and institutional affiliations.
Rights and permissions
Open Access This article is licensed under a Creative Commons Attribution 4.0 International License, which permits use, sharing, adaptation, distribution and reproduction in any medium or format, as long as you give appropriate credit to the original author(s) and the source, provide a link to the Creative Commons licence, and indicate if changes were made. The images or other third party material in this article are included in the article's Creative Commons licence, unless indicated otherwise in a credit line to the material. If material is not included in the article's Creative Commons licence and your intended use is not permitted by statutory regulation or exceeds the permitted use, you will need to obtain permission directly from the copyright holder. To view a copy of this licence, visit http://creativecommons.org/licenses/by/4.0/.
About this article
Cite this article
Yuan, Y., Yu, K., Zhang, C. et al. Generation of Textured Surfaces by Vibration-Assisted Ball-End Milling. Nanomanuf Metrol 6, 19 (2023). https://doi.org/10.1007/s41871-023-00198-2
Received:
Revised:
Accepted:
Published:
DOI: https://doi.org/10.1007/s41871-023-00198-2