Abstract
This study explores the transformative potential of Pillared InterLayered Clays (PILC) derived from non-conventional aluminum sources as catalytic supports in the synthesis of TiO2/catalysts for the efficient photodegradation of organic pollutants in water. Montmorillonite (Mt) and three alumina-pillared montmorillonite (PILC) synthesized using various aluminum sources, were impregnated with titanium to synthesize TiO2/catalysts. The successful synthesis of these materials was confirmed through several characterization techniques such as X-ray diffraction (XRD), N2 adsorption-desorption at -196 ºC, morphological analysis using scanning electron microscopy (SEM) and transmission electron microscopy (TEM), and Energy-Dispersive X-ray Spectrometry (EDX). The photolysis, adsorption, and catalytic behavior of the TiO2/catalysts were studied for the degradation of triclosan (TCS), 2,6-dichlorophenol (2,6-DCP), and bisphenol A (BPA). All synthesized catalysts surpassed the efficacy of commercial anatase, with TiO2/Al-PILC exhibiting superior performance in comparison to TiO2/Mt. Photodegradation was most effective under UV radiation, with TCS demonstrating the highest degradation (approximately 70%). Notably, Al-PILC samples, particularly those synthesized from saline slags, displayed enhanced properties. Among them, TiO2/Al-PILCAE exhibited the highest degradation rates under both UV and visible light, underlining the remarkable potential of saline slags as precursors for Al-PILC synthesis. This study provides valuable insights into the design and development of efficient catalysts for water treatment applications, paving the way for sustainable and effective solutions in the realm of environmental remediation.
Similar content being viewed by others
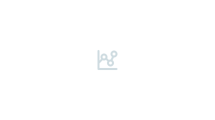
Explore related subjects
Discover the latest articles, news and stories from top researchers in related subjects.Avoid common mistakes on your manuscript.
1 Introduction
Among clays, smectites like montmorillonite (Mt) have been modified for various applications, including for the removal of organic compounds from water [1,2,3]. To address the problems related to their inherent characteristics and tendency to collapse at the layers, one effective modification has been made replacing the exchangeable cations within the interlayer space with large polyoxocations. This process forms stable structures or pillars, transforming the clay into a two-dimensional microporous solid, known as pillared interlayered clays (PILC). This modification enhances their stability and applicability significantly [1, 3, 4]. The superior pore volumes, basal spacings, and specific surface areas of PILC, compared to raw clays, broaden their potential applications. These enhanced properties and consistent interlayer and interpillar spaces make them effective catalysts and adsorbents [3, 5].
PILC synthesis comprises two key steps: intercalation, where polymeric inorganic cations replace exchangeable cations in the clay interlayer space, and thermal treatment, transforming intercalated polycations into rigid metal oxide pillars like Al2O3 through dehydration and dihydroxylation processes [6,7,8,9]. The intercalant polycations in Al-PILC montmorillonite synthesis are commonly prepared through a rapid hydrolysis process, using the titration method that includes a base and dissolved Al(III) salts [10,11,12]. The most documented method implies adding NaOH solution dropwise into the aluminum chloride solution [7, 11, 13,14,15,16,17,18,19,20,21,22,23,24,25,26,27,28].
However, previous research [29] demonstrated that Al-PILC synthesized using non-conventional aluminum sources like saline slag instead of commercial aluminum chloride solution exhibited enhanced properties. These improved characteristics were reflected in their performance as adsorbents for organic compounds [29, 30]. Highlighting that aluminum extraction from saline slag through alkaline and acid treatments offers potential for high-value products. Saline slag is a by-product of secondary aluminum production, is primarily composed of oxides, metallic aluminum, and salt residues [31,32,33]. The increasing production of this waste has led to environmental and economic challenges [32, 34, 35].
On the other hand, various methods have been studied to remove organic pollutants from water. Physical methods often preserve pollutants, maintaining their toxicity [3, 36], driving the interest in techniques like Advanced Oxidation Processes (AOP). AOP, including catalytic photodegradation, has been successfully used to remove pollutants from water [37, 38]. TiO2, a commonly used photocatalyst, offers advantages like non-toxicity, stability, and low cost [2, 39, 40]. However, its efficiency under visible light is limited due to its large band gap [41]. Despite this limitation, TiO2 has demonstrated selective degradation of certain organic compounds, such as chlorophenols and catechols, under visible radiation [42, 43]. To enhance TiO2 photocatalysis, supported nanostructured TiO2 catalysts have been explored, with porous solids like clays serving as effective catalyst supports. These modifications aim to improve photocatalytic efficiency and address challenges like post-separation of suspended TiO2 from treated water [44, 45]. TiO2 can be incorporated into clays through methods like pillaring or dispersed oxides formation via impregnation, precipitation, or sol-gel techniques [9, 46,47,48,49]. Both Ti-PILC and TiO2-nanocomposites have been effective in photocatalytic degradation, especially for dyes [9, 46, 48, 50,51,52]. The use of PILC instead of clays in the synthesis of TiO2-nanocomposite is a promising but underexplored approach due to the enhanced properties of PILC.
This study explores the removal of three priority hazardous phenolic compounds (PC) - Triclosan (TCS), 2,6-dichlorophenol (2,6-DCP), and bisphenol A (BPA) - from water using UV and VIS radiation together with TiO2 catalysts. The novelty of this work lies in demonstrating the potential of PILC as catalytic supports in the synthesis of TiO2-nanocomposites for organic pollutant photodegradation, without the use of hazardous oxidizing agents. Additionally, the study highlights the enhanced properties of Al-PILC obtained from alternatives aluminum sources, such as saline slags, which are significant waste products from secondary aluminum production and are considered hazardous.
2 Experimental Procedure
2.1 Materials and Reagents
The clay used as raw material in this study was montmorillonite clay from Tsukinuno, provided by The Clay Science Society of Japan. Two distinct aluminum sources were utilized in the synthesis of the aluminum polycation essential for the preparation of Al-PILC: saline slag waste obtained from Iberica de Aleaciones Ligeras S.L. (IDALSA) and aluminum chloride (AlCl₃·6H2O - PanReac AppliChem). The three Al-PILC evaluated as catalytic support in this study were synthesized through the conventional dropwise hydrolysis method. Sodium hydroxide (NaOH - PanReac AppliChem), and Hydrochloric acid (HCl 37% - PanReac AppliChem) were employed in both the extraction processes and the pH adjustment during the PILC synthesis.
The aqueous solutions of the organic compounds evaluated: 2,6-DCP (2,6-Dichlorophenol - C6H4Cl2O, Sigma Aldrich), BPA (Bisphenol A − 4,4’-(propane-2,2-diyl)diphenol - C15H16O2, Sigma Aldrich), and TCS (Triclosan − 5-chloro-2-(2,4-dichlorophenoxy)phenol - C12H7Cl3O2, Alfa Aesar) were prepared with ultrapure water (Milli-Q apparatus - Millipore) and ethanol (PanReac AppliChem) to improve their solubility. The photodegradation of the three organic compounds was compared under identical conditions using the commercial titanium(IV) oxide form (anatase, > 99%, Sigma-Aldrich).
2.2 Aluminum Extraction
Aluminum extraction from the aluminum saline slag was carried out using alkaline or acid solutions. In each case, the saline slag underwent reflux for 2 h (0.75 dm3 of 2 mol/dm3 aqueous solutions of NaOH or HCl). The resulting slurries were separated through centrifugation (Hettich - Rotanta 460 S). The aluminum content quantified using ICP-OES in the solutions was 9.24 g/cm3 (alkaline) and 9.37 g/dm3 (acid).
2.3 Synthesis of the TiO2/Al-PILC Catalysts
Initially, the synthesis of the three Al-PILC catalytic supports was carried out through the previously mentioned conventional dropwise hydrolysis method. One of these supports, referred to as Al-PILCCM, was synthesized using aluminum chloride (AlCl₃.6H2O) as the aluminum source. The hydrolysis reaction with NaOH was conducted using a molar ratio of [ОН–]/[Аl3+] set as 2. The remaining two supports were synthesized utilizing saline slag as the aluminum source. One was synthesized from the acid extract of the slag, designated as Al-PILCAE, while the other was from the alkaline extract, named Al-PILCBE. Their synthesis involved specific conditions optimized for each case in previous research [29], including a [ОН–]/[Аl3+] molar ratio of 2.0 for both alkaline and acid aluminum extracts, Al/clay (mmol/g) ratio of 20 (alkaline) and 7 (acid), clay/volume solution ratio of 8 (alkaline) and 6 (acid), and the titration process carried out at room temperature (alkaline) and above 90 °C (acid).
The intercalation time was 22 h at room temperature. Upon completion, the samples were separated from the solution through centrifugation (Hettich - Rotanta 460 S), washed multiple times with distilled water, and subsequently dried (at 100 °C for 8 h). Following drying, the samples underwent calcination in an oven (Nabertherm L5/S27) at 500 °C for 4 h, with a heating rate of 1 °C per minute.
TiO2/Mt and TiO2/Al-PILC were synthesized via wet impregnation of the four dried catalytic supports, which were dried at 150 °C for 12 h: raw clay (Mt), as well as the three Al-PILC (Al-PILCCM, Al-PILCBE, and Al-PILCAE). This process was carried out using titanium(IV) isopropoxide and isopropyl alcohol and was followed by calcination [53]. The desired percentage of Ti (5 and 20 wt%) in the catalyst was achieved by carefully dosing the appropriate amount of the doping agent throughout the process.
The impregnated solids were dried to eliminate the solvent, involving heating at 100 °C for 8 h and then at 150 °C for 12 h. Following this, the solids were calcined at 500 °C for 4 h, with a heating rate of 1 °C per minute. These parameters were carefully chosen, considering both the calcination temperature suitable for the supports and the temperature required for the formation of the anatase form.
2.4 Characterization of the TiO2/Catalysts
The solids were characterized by XRD (powder X-ray diffraction) with Ni-filtered Cu Kα radiation (λ = 0.1548 nm) on a BRUKER D-8 ADVANCE eco X-ray diffractometer. Spectra were obtained at a scan rate of 0.2°(2θ)/min in a range from 2° to 20° or 70° (2θ) for the catalytic supports, and from 8 to 70° (2θ) for the TiO2 catalysts. Basal spacings were determined based on the corresponding basal reflections’ 2θ values.
The textural properties of the samples were assessed through N2 adsorption experiments conducted at -196 °C, using a gas sorption analyzer (Micromeritics ASAP 2020 Plus) and high-purity N2 gas (Praxair, 99.999%). Prior to analysis, the samples (0.2 g) underwent a degassing process at 200 °C for 12 h under a pressure lower than 0.1 Pa. The specific surface area (SBET) was estimated applying the Brunauer–Emmett–Teller (BET) method in the relative pressure range from 0.05 to 0.2, while the total pore volume (VTotal) was calculated based on the amount of nitrogen adsorbed at a relative pressure of 0.98.
Finally, the morphology of the catalytic supports and the TiO2/catalysts, as their chemical composition were studied using Scanning Electron Microscopy (SEM) and Energy-Dispersive X-ray Spectrometry (EDX) with an INSPECT- F50 at 10 kV. Additionally, Transmission Electron Microscopy (TEM) was conducted using a Tecnai T30 transmission electron microscope operated at an accelerating voltage of 200 kV.
2.5 Photodegradation Experiments
All the photocatalytic (UV or VIS radiation), photolysis (absence of a catalyst) and adsorption (absence of light) experiments were carried out at room temperature (24 °C). Photocatalytic experiments were conducted inside a photon cabinet, using a 1.0 dm3 PhotoLAB Batch-L reactor (MPDS®Basic system from UV-Consulting Peschl, Spain). The set up included a water-cooling jacket (PolyScience Digital Temperature, model 9702) ensuring a constant temperature of 24 °C.
The lamp was positioned at the center of the reactor. UV tests used a medium-pressure Hg lamp (TQ 150Z1 - power 150 W) with peaks at 366 nm (Φ = 6.4 W) and 313 nm (Φ = 4.3 W) while visible tests used a TXe-150 Xenon lamp with 312 nm cutoff filter.
The study initially focused on investigating the effect of pH. Aqueous solutions (0.7 dm3) containing the organic pollutants (TCS, 2,6-DCP, or BPA) at a concentration of 25 µmol/dm3 were adjusted to specific pH levels (ranging from 2 to 10) by adding either HCl or NaOH. commercial anatase was employed as a catalyst, added to the respective solution in the reactor (200 mg), and continuously stirred at 700 rpm under UV radiation. Degradation efficiency was monitored at specified intervals using a spectrometer (Jasco V-730 UV–VIS) by tracking absorption changes in accordance with the Beer–Lambert law. Samples taken at designated time points were filtered to remove the catalyst (Durapore membrane filters, 0.45 μm) before measuring absorption at the maximum absorption wavelength for each of organic compound: 279.4 nm (TCS), 282.6 nm (2,6-DCP), and 276.2 nm (BPA). After identifying the pH range that yielded superior degradation, the experiment was replicated using TiO2/Al-PILC based catalysts.
The photodegradation experiments utilized the catalysts in aqueous solutions at two initial pollutant concentrations (60 and 90 µmol/dm3), with varying catalyst amounts (200 and 350 mg) in each case. Concurrently, adsorption experiments, conducted in darkness, were performed using the highest pollutant concentration (90 µmol/dm3) and the maximum catalyst dosage (350 mg) from the photodegradation experiments. These experiments facilitated a comparative analysis, shedding light on the effect of adsorption in the pollutant removal process. The photodegradation experiments were replicated, substituting the catalyst with commercial anatase for comparative analysis.
3 Results and Discussion
3.1 Characterization of Catalytic Supports and TiO2/Catalysts
The basal spacings of the four catalytic supports (Montmorillonite and the three Al-PILC variants) were determined through XRD analysis. After the intercalation process, Montmorillonite (Mt) exhibits a broader basal spacing, indicated by the d001 towards the lower 2θ region in the XRD diffractogram, as illustrated in Fig. 1. The basal spacing of the PILC were 1.85 nm (Al-PILCCM), 1.78 nm (Al-PILCBE), and 1.94 nm (Al-PILCAE), confirming the successful pillaring of Mt (9.9 nm). The findings concerning the specific surface area (SBET), total pore volume (VTotal), and the average pore diameter (dp) of the four catalytic supports are presented in Table 1. The results suggest that both aluminum extracts are suitable for synthesizing Al-PILC. However, the values clearly show that the PILC with larger basal spacing and specific surface areas is obtained when using the aluminum acid extract from the saline slag as the aluminum source.
Figure 2 displays the XRD patterns of the catalysts (TiO2/Mt and TiO2/Al-PILC), revealing distinct diffraction peaks associated with the anatase phase (JCPDS file 78-2486), as depicted in the figure. The crystallite size of TiO2 particles in all samples was calculated using the Debye–Scherer equation (Eq. 1) [38], utilizing the most intense diffraction peak (101) of the anatase phase (25.55°) in the XRD patterns.
In the equation, D represents the crystal size (in nanometers), β stands for the width of the anatase peak at half maximum (FWHM), θ denotes the Bragg angle, K is the crystallite shape factor (0.89), and λ represents the X-ray wavelength.
The crystal size of TiO2 in the catalysts impregnated with 5 and 20 wt% titanium ranged from 14.36 to 24.81 (Mt), 14.37 and 22.92 nm (Al-PILCAE), 16.40 and 21.84 nm (Al-PILCBE), and 13.95 and 25.48 nm (Al-PILCCM). These sizes, consistent with findings from other researchers [53, 54], significantly exceed the previously mentioned basal spacings This suggests that the majority of TiO2 is located on the external surface of the Al-PILC. With Mt, there was a noticeable increase in the SBET area as the titanium loading increased, consistent with prior studies on TiO2/Mt nanocomposites [5, 55]. This trend contrasts with the behavior observed in PILC. These differences highlight the variations in structural and morphological characteristics between the two materials and underscore how these distinctions can impact their roles as catalytic supports.
Figure 3 shows the N2 adsorption-desorption isotherms for both the catalytic supports (A) and the catalysts at 20 wt% Ti (B). The adsorption isotherms exhibit characteristics typical of Al-PILC and are classified as Type II according to the IUPAC classification [56]. The wide range of pore sizes is evident from the hysteresis loop observed in the isotherms [54]. Total pore volume (VTotal), specific surface area (SBET), and average pore diameter (dp) of the catalysts are detailed in Table 1, providing insight into their textural properties.
Figure 4 provides an insight into the morphological features of both catalytic supports and TiO2/catalysts through SEM micrographs and representative TEM images. SEM images reveal distinct surface morphologies between Mt and Al-PILC. The comparison between 0% and 20% titanium contents highlights the morphological distinctions between the solid support used for the catalyst and the synthesized catalysts. In the case of Mt, a comparison of the micrographs underscores a significant alteration in its ordered and layered structure upon the incorporation of TiO2, revealing the presence of disordered and exfoliated structures, including curled plates. These findings align with results reported by other researchers [48]. The micrographs demonstrate noticeable alterations in the surface morphology of the three Al-PILC samples upon the incorporation of TiO2. Both SEM and TEM images suggest the presence of TiO2 particles on the external surface of the catalyst supports, a phenomenon in line with observations reported by other researchers [54]. The size of these TiO2 particles ranges from 9.10 to 23.22 nm (Mt with 5 and 20 wt% Ti), 6.73 to 22.01 nm (Al-PILCAE with 5 and 20 wt% Ti), 7.15 to 22.51 nm (Al-PILCBE with 5 and 20 wt% Ti), and 7.61 to 23.12 nm (Al-PILCCM with 5 and 20 wt% Ti). In all cases, most of the measured particles are concentrated around their respective maximum values. These results are consistent with those of both the XRD and N2 adsorption.
Additionally, EDS analysis confirms the presence of TiO2 in all samples, as detailed in Table 2. The values provided in the table also support the successful control of titanium contents in the TiO2 catalysts. This is evident from the close match between the experimental titanium content in the samples and the theoretical one used in the impregnation process. The relative error ranged from 7.3% (Al-PILCAE) to 13.5% (Al-PILCCM).
3.2 Photocatalytic Experiments
The highest photodegradation occurred within the pH range of 3–5 for all compounds, with TCS showing the maximum degradation of 14.25% (2,6-DCP), 28.18% (TCS) y 13.32% (BPA). From these results, the TiO2/catalysts were evaluated specifically within this pH range. The results depicted in Fig. 5. clearly demonstrate the pH-dependent photodegradation of the three pollutants. Within the acidic pH range, elevating the initial pH enhanced pollutant degradation until reaching their respective maximum degradation points. Beyond these points, further increase in initial pH led to a significant decrease in degradation efficiency. Notably, a pH of 4.0 exhibited the highest degradation rates for the organic pollutants, prompting its selection for subsequent experiments. This value aligns with the pH values employed by other researchers [57, 58].
The photodegradation experiments were conducted as detailed in the experimental section. For each experiment, the ratio Ct/Co (where Ct represents the concentration at time t and Co is the initial concentration) was plotted against reaction time. The experiments started with initial photolysis tests, involving the degradation of the three organic pollutants (90 µmol/dm3) under both UV and VIS radiation in the absence of any catalyst. Figure 6 shows the photolysis plots for the organic pollutants. These experiments revealed that under UV radiation, 2,6-DCP and TCS exhibited photodegradation rates of approximately 14% and 38%, respectively, whereas under VIS radiation, the degradation rates dropped significantly to 5% for 2,6-DCP and 6% for TCS. In contrast, BPA showed minimal photodegradation, ranging from 0.39 to 0.89%, consistent with previous studies that highlighted the limited photolysis of BPA during short-term irradiation [59, 60].
In the adsorption experiments, BPA demonstrated the lowest adsorption rates (< 1%), followed by 2,6-DCP (< 10%), and TCS that exhibited comparatively higher adsorption rates (< 30%). The removal efficiency of all catalysts for the three pollutants was significantly higher when impregnated at 20 wt% Ti compared to 5 wt% Ti, emphasizing the crucial role of titanium content in the photodegradation process. Figure 7 illustrates the UV-degradation of TCS and 2,6-DCP, the pollutants with the highest photodegradation rates, employing TiO2/catalysts with 20 wt% Ti. All pollutants exhibited the highest removal rates at the higher concentration (90 µmol/dm3) and when utilizing the maximum catalyst dose (350 mg). This underscores the significance of both the catalytic amount and the solution concentration in the removal process. At both titanium impregnation levels (5 and 20 wt% Ti), the three Al-PILC catalysts demonstrated higher photodegradation percentages for all three pollutants compared to TiO2/Mt. Among the Al-PILC variants, Al-PILCAE exhibited the highest photodegradation values for the pollutants. These differences can be attributed to the properties and morphologies of these materials, including the dispersion and size of TiO2 particles on their surfaces. Smaller TiO2 crystals, particularly those smaller than 10 nm, have been reported to exhibit superior catalytic performance [49]. Hence, the presence of smaller particles on the catalyst’s surface likely plays a significant role in the obtained photocatalytic results. Additionally, the role of adsorption is crucial [53]; higher photodegradation rates are achieved when the adsorption of the respective pollutant on the catalyst is more significant. This is because pollutants need to be adsorbed on the catalyst’s surface before the photodegradation reaction can take place. The interplay between adsorption and photodegradation accelerates the photocatalytic process, possibly due to the presence of photoactive TiO2 particles. Although quantifying the exact contribution of adsorption to pollutant removal is challenging, it is evident that the catalysts employed rely on a combination of adsorption and photodegradation to effectively remove pollutants from water, particularly the chlorophenols studied in this work.
The pollutant most significantly photodegraded was TCS (approximately 70%), followed by 2,6-DCP (around 40%), and, to a lesser extent, BPA (approximately 22%). The photodegradation of organic compounds was consistently higher in all cases under UV radiation. A comparative removal efficiency for the three organic compounds using both types of radiation is depicted in a stacked column analysis in Fig. 8. The comparison between the amounts eliminated using UV and VIS light in this graphic for each pollutant reveals a smaller difference for 2,6-DCP. This exhibits that 2,6-DCP experienced higher photodegradation using VIS radiation, with approximately 66–77% of the degradation achieved under UV radiation. The higher elimination rates of 2,6-DCP and TCS compared to BPA under VIS radiation align with findings from other studies, indicating that chlorophenols are more susceptible to photodegradation by TiO2 using visible radiation [42, 43].
Comparative Removal Efficiency (Normalized) of TCS, 2,6-DCP, and BPA by Four Catalysts (A) TiO2/Mt, (B)TiO2/Al-PILCBE, (C) TiO2/Al-PILCAE, and (D) TiO2/Al-PILCCM (catalysts at 20 wt% Ti): Stacked Column Analysis Under UV and Visible Radiation. Experimental Conditions: Solutions at 90 µmol/dm3 and 350 mg catalysts used
The kinetic constants of the experiments were calculated based on a first-order rate equation under conditions of higher degradation rates: utilizing 350 mg of catalysts and a pollutant solution of 90 µmol/dm³. These values are summarized in Table 3, which also includes calculations under the same conditions for commercial anatase and without any catalyst (photolysis). The titanium content in anatase is approximately 60%, making direct comparisons challenging even with the same catalyst dose, given that the catalysts under study contain a maximum of 20 wt% titanium. To enable a fair comparison, experiments were conducted using anatase in quantities equivalent to the same titanium content. None of these experiments led to the degradation of any of the three pollutants.
4 Conclusions
In this work, montmorillonite (Mt) and three alumina-pillared montmorillonite (PILC) synthesized using various aluminum sources, were impregnated with titanium to synthesize TiO2/catalysts. The materials were thoroughly characterized through several characterization techniques such as XRD, N2 adsorption experiments at -196 ºC and morphological analysis using scanning electron microscopy (SEM) and transmission electron microscopy (TEM). Photolysis, adsorption, and catalytic behavior for the photodegradation of triclosan (TCS), 2,6-dichlorophenol (2,6-DCP), and bisphenol A (BPA) were studied. The results showed that all the catalysts exhibited higher catalytic performance than commercial anatase, with TiO2/Al-PILC demonstrating superior performance compared to TiO2/Mt. Photodegradation was more significant under UV radiation, and TCS exhibited the highest photodegradation (approximately 70%), followed by 2,6-DCP (around 40%) and BPA (approximately 22%). The role of adsorption in pollutant removal was crucial, indicating that the catalysts relied on a combination of adsorption and photodegradation for efficient pollutant removal. Al-PILC, especially those synthesized using saline slags as the aluminum source, demonstrated enhanced properties, with the TiO2/Al-PILCAE catalyst exhibiting the highest degradation rates under both UV and visible radiation. These results emphasize the potential of saline slags as precursors for Al-PILC synthesis and the effectiveness of these materials in pollutant removal from water. The TiO2/catalysts demonstrated superior performance, with Al-PILCAE exhibiting the highest photodegradation rates for the pollutants studied. Overall, the findings highlight the potential of PILC as catalytic supports in TiO2-nanocomposites for organic pollutant photodegradation and underscore the importance of titanium content and catalyst morphology in enhancing catalytic efficiency.
Data Availability
Hazardous wastes list, 100308.
References
González-Rodríguez B, Trujillano R, Rives V, Vicente MA, Gil A, Korili SA (2015) Structural, Textural and Acidic properties of Cu-, Fe- and Cr-Doped Ti-Pillared montmorillonites. Appl Clay Sci 118:124–130. https://doi.org/10.1016/j.clay.2015.09.010
González B, Trujillano R, Vicente MA, Rives V, de Faria EH, Ciuffi KJ, Korili SA, Gil A (2017) Doped Ti-Pillared Clays as effective adsorbents – application to Methylene Blue and Trimethoprim removal. Environ Chem 14:267–278. https://doi.org/10.1071/EN16192
Cardona Y, Vicente MA, Korili SA, Gil A (2022) Progress and perspectives for the Use of Pillared Clays as adsorbents for Organic compounds in Aqueous Solution. Rev Chem Eng 38. https://doi.org/10.1515/revce-2020-0015
Cardona Y, Korili SA, Gil A (2021) Understanding the formation of Al13 and Al30 polycations to the development of Microporous materials based on Al13-and Al30-PILC montmorillonites: a review, Appl. Clay Sci 203:105996. https://doi.org/10.1016/j.clay.2021.105996
Cardona Y, Węgrzyn A, Miśkowiec P, Korili SA, Gil A (2022) Catalytic Photodegradation of Organic compounds using TiO2/Pillared clays synthesized using a nonconventional aluminum source. Chem Eng J 446:136908. https://doi.org/10.1016/j.cej.2022.136908
Nunes CD, Pires J, Carvalho AP, Calhorda MJ, Ferreira P (2008) Synthesis and characterisation of Organo-Silica Hydrophobic Clay Heterostructures for volatile Organic compounds removal. Microporous Mesoporous Mater 111:612–619. https://doi.org/10.1016/j.micromeso.2007.09.008
Zhu J, Wen K, Wang Y, Ma L, Su X, Zhu R, Xi Y, He H (2018) Superior Thermal Stability of Keggin-Al30 Pillared Montmorillonite: a comparative study with Keggin-Al13 Pillared Montmorillonite. Microporous Mesoporous Mater 265:104–111. https://doi.org/10.1016/j.micromeso.2018.02.007
Gil A, Gandía LM, Vicente MA (2000) Recent advances in the synthesis and Catalytic Applications of Pillared Clays. Catal Rev 42:145–212. https://doi.org/10.1081/CR-100100261
Yuan P, Yin X, He H, Yang D, Wang L, Zhu J (2006) Investigation on the delaminated-pillared structure of TiO2-PILC synthesized by TiCl4 hydrolysis method. Microporous Mesoporous Mater 93:240–247. https://doi.org/10.1016/j.micromeso.2006.03.002
Mertens J, Casentini B, Masion A, Pothig R, Wehrli B, Furrer G (2012) Polyaluminum Chloride with high Al30 content as removal Agent for Arsenic-Contaminated Well Water. Water Res 46:53–62. https://doi.org/10.1016/j.watres.2011.10.031
Corum KW, Fairley M, Unruh DK, Payne MK, Forbes TZ, Mason SE (2015) Characterization of phosphate and Arsenate Adsorption onto Keggin-Type Al30 cations by experimental and theoretical methods. Inorg Chem 54:8367–8374. https://doi.org/10.1021/acs.inorgchem.5b01039
Fairley M, Unruh DK, Abeysinghe S, Forbes TZ (2012) Synthesis and structural characterization of Heterometallic Thorium Aluminum Polynuclear Molecular clusters. Inorg Chem 51:9491–9498. https://doi.org/10.1021/ic3013014
Casey WH, Molecules LAAH (2006) Chem Rev 106:1–16. https://doi.org/10.1021/cr040095d
Allouche L, Taulelle F (2003) Fluorination of the ε-Keggin Al13 polycation. Chem Commun 2084–2085. https://doi.org/10.1039/B303585A
Allouche L, Gérardin C, Loiseau T, Férey G, Taulelle F (2000) Al30: a giant aluminum polycation, Angew. Chemie Int Ed 39:511–514. https://doi.org/10.1002/(SICI)1521-3773(20000204)39:3%3C511::AID-ANIE511%3E3.0.CO;2-N
Abeysinghe S, Unruh DK, Forbes TZ (2013) Surface modification of Al30 keggin-type polyaluminum molecular clusters. Inorg Chem 52:5991–5999. https://doi.org/10.1021/ic400321k
Cheng LS, Yang RT (1997) Tailoring micropore dimensions in Pillared Clays for enhanced gas adsorption. Microporous Mater 8:177–186. https://doi.org/10.1016/S0927-6513(96)00067-3
Gil A, Vicente MA, Gandía LM (2000) Main factors Controlling the texture of Zirconia and Alumina Pillared Clays. Microporous Mesoporous Mater 34:115–125. https://doi.org/10.1016/S1387-1811(99)00166-3
Parker WO Jr., Millini R, Kiricsi I (1997) Metal substitution in Keggin-Type Tridecameric Aluminum-Oxo-Hydroxy clusters. Inorg Chem 36:571–575. https://doi.org/10.1021/ic960635s
Guerra DL, Airoldi C, Lemos VP, Angélica RS (2008) Adsorptive, Thermodynamic and Kinetic performances of Al/Ti and Al/Zr-Pillared Clays from the Brazilian Amazon Region for Zinc Cation removal. J Hazard Mater 155:230–242. https://doi.org/10.1016/j.jhazmat.2007.11.054
Gil A, Montes M (1994) Effect of Thermal Treatment on Microporous accessibility in Aluminium Pillared Clays. J Mater Chem 4:1491–1496. https://doi.org/10.1039/JM9940401491
Gil A, Korili SA, Vicente MA (2008) Recent advances in the control and characterization of the porous structure of Pillared Clay catalysts. Catal Rev 50:153–221. https://doi.org/10.1080/01614940802019383
Wen K, Wei J, He H, Zhu J, Xi Y (2019) Keggin-Al30: an intercalant for Keggin-Al30 Pillared Montmorillonite. Appl Clay Sci 180:105203. https://doi.org/10.1016/j.clay.2019.105203
Zhu J, Wen K, Zhang P, Wang Y, Ma L, Xi Y, Zhu R, Liu H, He H (2017) Keggin-Al30 Pillared Montmorillonite. Microporous Mesoporous Mater 242:256–263. https://doi.org/10.1016/j.micromeso.2017.01.039
Bradley SM, Kydd RA, Yamdagni R (1990) Comparison of the hydrolyses of Gallium(III) and Aluminium(III) solutions by nuclear magnetic resonance spectroscopy. J Chem Soc Dalt Trans 2653–2656. https://doi.org/10.1039/DT9900002653
Zuo S, Zhou R (2008) Influence of Synthesis Condition on Pore structure of Al Pillared Clays and supported pd catalysts for deep oxidation of Benzene. Microporous Mesoporous Mater 113:472–480. https://doi.org/10.1016/j.micromeso.2007.12.005
Pesquera C, Gonzalez F, Benito I, Mendioroz S, Pajares JA (1991) Synthesis and characterization of Pillared Montmorillonite catalysts. Appl Catal 69:97–104. https://doi.org/10.1016/S0166-9834(00)83294-7
Hutson ND, Hoekstra MJ, Yang RT (1999) Control of Microporosity of Al2O3-Pillared Clays: Effect of pH, calcination temperature and clay Cation Exchange Capacity. Microporous Mesoporous Mater 28:447–459. https://doi.org/10.1016/S1387-1811(98)00334-5
Cardona Y, Korili SA, Gil A (2021) A nonconventional aluminum source in the Production of Alumina-Pillared Clays for the removal of Organic pollutants by Adsorption. Chem Eng J 425:130708. https://doi.org/10.1016/j.cej.2021.130708
Cardona Y, Korili SA, Gil A (2023) Use of response surface methodology to optimize triclosan adsorption on alumina pillared clays in a fixed-bed column for applications in solid-phase extraction. Appl Clay Sci 235:106879. https://doi.org/10.1016/j.clay.2023.106879
Gil A (2005) Management of the Salt cake from secondary Aluminum Fusion processes. Ind Eng Chem Res 44:8852–8857. https://doi.org/10.1021/ie050835o
Gil A, Korili SA (2016) Management and valorization of aluminum saline slags: current Status and Future trends. Chem Eng J 289:74–84. https://doi.org/10.1016/j.cej.2015.12.069
Verma SK, Dwivedi VK, Dwivedi SP (2021) Utilization of Aluminium Dross for the Development of Valuable Product – A Review, Mater. Today Proc. https://doi.org/10.1016/j.matpr.2020.12.045
Mahinroosta M, Allahverdi A, Critical Review (2018) J Environ Manage 223:452–468. https://doi.org/10.1016/j.jenvman.2018.06.068
Meshram A, Singh KK (2018) Recovery of Valuable products from Hazardous Aluminum Dross: a review, Resour. Conserv Recycl 130:95–108. https://doi.org/10.1016/j.resconrec.2017.11.026
Dai M, Luo Z, Luo Y, Zheng Q, Zhang B (2022) Degradation of 2,6-dichlorophenol by Ferrate (VI) oxidation: kinetics, performance, and mechanism. Sep Purif Technol 278:119475. https://doi.org/10.1016/j.seppur.2021.119475
Cardona Y, Węgrzyn A, Miśkowiec P, Korili SA, Gil A (2023) Heterogeneous Fenton- and photo-Fenton-like catalytic degradation of emerging pollutants using Fe2O3/TiO2/pillared clays synthesized from aluminum industrial wastes. J Water Process Eng 52:103494. https://doi.org/10.1016/j.jwpe.2023.103494
Cardona Y, Korili SA, Gil A Use of clays and pillared clays in the catalytic photodegradation of organic compounds in aqueous solutions. Catal Rev (n d) 1–48. https://doi.org/10.1080/01614940.2023.2178736
Norabadi E, Ashrafi SD, Kamani H, Jahantiq A (2020) Degradation of 2,6-dichlorophenol by Fe-Doped TiO2 Sonophotocatalytic process: kinetic study, Intermediate product, degradation pathway. Int J Environ Anal Chem 1–16. https://doi.org/10.1080/03067319.2020.1837122
Torres RA, Pétrier C, Combet E, Moulet F, Pulgarin C (2007) Bisphenol a mineralization by Integrated Ultrasound-UV-Iron (II) treatment. Environ Sci Technol 41:297–302. https://doi.org/10.1021/es061440e
Ghrib T, AL-Saleem NK, AL-Naghmaish A, Elshekhipy AA, Brini S, Briki K, Elsayed KA (2021) Annealing effect on the Microstructural, Optical, Electrical, and Thermal properties of Cu2O/TiO2/Cu2O/TiO2/Si Heterojunction prepared by Sol-Gel Technique, Superlattices Microstruct. 107119. https://doi.org/10.1016/j.spmi.2021.107119
Paul T, Miller PL, Strathmann TJ (2007) Visible-light-mediated TiO2 photocatalysis of Fluoroquinolone Antibacterial agents. Environ Sci Technol 41:4720–4727. https://doi.org/10.1021/es070097q
Jiang Y, Qin Y, Yu T, Lin S (2021) Synthesis of Sponge-Like TiO2 with surface-phase junctions for enhanced visible-light photocatalytic performance. Chin Chem Lett 32:1823–1826. https://doi.org/10.1016/j.cclet.2020.11.010
Joy VM, Dutta S, Feroz S, Devi G (2021) Nanophotocatalytic treatment of seawater using TiO2 immobilized and suspension system under solar irradiation. J Water Process Eng 43:102263. https://doi.org/10.1016/j.jwpe.2021.102263
Gil A, García AM, Fernández M, Vicente MA, González-Rodríguez B, Rives V, Korili SA (2017) Effect of dopants on the structure of Titanium Oxide used as a Photocatalyst for the removal of Emergent contaminants. J Ind Eng Chem 53:183–191. https://doi.org/10.1016/j.jiec.2017.04.024
González B, Trujillano R, Vicente MA, Rives V, Korili SA, Gil A (2019) Photocatalytic degradation of Trimethoprim on Doped Ti-Pillared Montmorillonite. Appl Clay Sci 167:43–49. https://doi.org/10.1016/j.clay.2018.10.006
Ding Z, Zhu HY, Lu GQ, Greenfield PF (1999) Photocatalytic Properties of Titania Pillared Clays by different drying methods. J Colloid Interface Sci 209:193–199. https://doi.org/10.1006/jcis.1998.5857
Miao S, Liu Z, Han B, Zhang J, Yu X, Du J, Sun Z (2006) Synthesis and characterization of TiO2–Montmorillonite nanocomposites and their application for removal of Methylene Blue. J Mater Chem 16:579–584. https://doi.org/10.1039/B511426H
Zhu HY, Orthman JA, Li J-Y, Zhao J-C, Churchman GJ, Vansant EF (2002) Novel composites of TiO2 (Anatase) and Silicate Nanoparticles. Chem Mater 14:5037–5044. https://doi.org/10.1021/cm0205884
Kočí K, Matějka V, Kovář P, Lacný Z, Obalová L (2011) Comparison of the pure TiO2 and Kaolinite/TiO2 Composite as Catalyst for CO2 photocatalytic reduction. Catal Today 161:105–109. https://doi.org/10.1016/j.cattod.2010.08.026
Ma J, Jia Y, Jing Y, Sun J, Yao Y (2009) Synthesis and photocatalytic activity of TiO2-Hectorite composites. Appl Clay Sci 46:114–116. https://doi.org/10.1016/j.clay.2009.07.011
Sahel K, Bouhent M, Belkhadem F, Ferchichi M, Dappozze F, Guillard C, Figueras F (2014) Photocatalytic Degradation of Anionic and Cationic dyes over TiO2 P25, and Ti-Pillared Clays and Ag-Doped Ti-Pillared Clays. Appl Clay Sci 95:205–210. https://doi.org/10.1016/j.clay.2014.04.014
Djellabi R, Ghorab MF, Cerrato G, Morandi S, Gatto S, Oldani V, Di Michele A, Bianchi CL (2014) Photoactive TiO2–Montmorillonite composite for degradation of Organic dyes in Water. J Photochem Photobiol Chem 295:57–63. https://doi.org/10.1016/j.jphotochem.2014.08.017
Deepracha S, Vibulyaseak K, Ogawa M (2019) Chap. 2.1 - Complexation of TiO2 with Clays and Clay Minerals for Hierarchically Designed Functional Hybrids, in: K. Ariga, M.B.T.-A.S.N. Aono (Eds.), Micro Nano Technol., William Andrew Publishing, : pp. 125–150. https://doi.org/10.1016/B978-0-12-813341-5.00010-3
Belessi V, Lambropoulou D, Konstantinou I, Katsoulidis A, Pomonis P, Petridis D, Albanis T (2007) Structure and photocatalytic performance of TiO2/Clay nanocomposites for the degradation of Dimethachlor. Appl Catal B Environ 73:292–299. https://doi.org/10.1016/j.apcatb.2006.12.011
Thommes M, Kaneko K, Neimark AV, Olivier JP, Rodriguez-Reinoso F, Rouquerol J, Sing KSW (2015) Physisorption of gases, with special reference to the evaluation of surface area and pore size distribution (IUPAC Technical Report). Pure Appl Chem 87:1051–1069. https://doi.org/10.1515/pac-2014-1117
Kansal SK, Chopra M (2012) Photocatalytic degradation of 2,6-Dichlorophenol in Aqueous Phase using Titania as a Photocatalyst, Engineering. 4 416–420. https://doi.org/10.4236/eng.2012.48055
Watanabe N, Horikoshi S, Kawabe H, Sugie Y, Zhao J, Hidaka H (2003) Photodegradation mechanism for Bisphenol A at the TiO2/H2O interfaces, Chemosphere. 52 851–859. https://doi.org/10.1016/S0045-6535(02)00837-8
Rosenfeldt EJ, Linden KG (2004) Degradation of endocrine disrupting Chemicals Bisphenol A, Ethinyl Estradiol, and Estradiol during UV photolysis and advanced oxidation processes. Environ Sci Technol 38:5476–5483. https://doi.org/10.1021/es035413p
Garg A, Singhania T, Singh A, Sharma S, Rani S, Neogy A, Yadav SR, Sangal VK, Garg N (2019) Photocatalytic degradation of Bisphenol-A using N, Co Codoped TiO2 Catalyst under Solar Light. Sci Rep 9:765. https://doi.org/10.1038/s41598-018-38358-w
Acknowledgements
The authors are grateful for financial support from the Spanish Ministry of Science and Innovation (MCIN/AEI/https://doi.org/10.13039/501100011033) through project PID2020-112656RBC21. YC thanks the Universidad Pública de Navarra for a pre-doctoral grant (IberusTalent, European Union’s H2020 research and innovation programme under Marie Sklodowska-Curie grant agreement N° 801586). Open access funding provided by Universidad Pública de Navarra.
Funding
No funding was received for this work.
Author information
Authors and Affiliations
Corresponding author
Ethics declarations
Ethical Approval
We further confirm that any aspect of the work covered in this manuscript that has involved human patients has been conducted with the ethical approval of all relevant bodies and that such approvals are acknowledged within the manuscript.
Competing Interests
Not applicable.
Additional information
Publisher’s Note
Springer Nature remains neutral with regard to jurisdictional claims in published maps and institutional affiliations.
Rights and permissions
Open Access This article is licensed under a Creative Commons Attribution 4.0 International License, which permits use, sharing, adaptation, distribution and reproduction in any medium or format, as long as you give appropriate credit to the original author(s) and the source, provide a link to the Creative Commons licence, and indicate if changes were made. The images or other third party material in this article are included in the article’s Creative Commons licence, unless indicated otherwise in a credit line to the material. If material is not included in the article’s Creative Commons licence and your intended use is not permitted by statutory regulation or exceeds the permitted use, you will need to obtain permission directly from the copyright holder. To view a copy of this licence, visit http://creativecommons.org/licenses/by/4.0/.
About this article
Cite this article
Cardona, Y., Gil, A. TiO2/Al-PILC Catalysts Synthesized from a Non-Conventional Aluminum Source of Aluminum and Applied in the Photodegradation of Organic Compounds. Top Catal (2024). https://doi.org/10.1007/s11244-024-01994-w
Accepted:
Published:
DOI: https://doi.org/10.1007/s11244-024-01994-w