Abstract
Background and Aims
Species diversity is expected to increase with environmental heterogeneity. For plant communities, this pattern has been confirmed by numerous observational studies. Yet, experimental studies yield inconsistent results potentially because of how experiments create soil heterogeneity or because seeds were sown homogeneously. Using a field experiment, we tested how soil heterogeneity, plant spatial aggregation via seed arrival, and grain size influence plant species richness in a restored grassland.
Methods
We manipulated soil heterogeneity and seed arrival in 0.2 × 0.2 or 0.4 × 04 m patches within each 4.0 × 4.6 m plot and allowed community assembly to occur for 4 growing seasons.
Results
Despite quantifiable soil differences, soil heterogeneity did not impact total or sown species richness, but did weakly influence non-sown richness. Richness differences were driven by non-sown plant species that likely exhibited higher establishment in aggregated plots due to decreased interspecific competition and conspecific facilitation.
Conclusion
Our results suggest that fine-scale soil heterogeneity weakly affects prairie plant diversity, but heterogeneous plant spatial structure can have a stronger effect on diversity. These results suggest that plant colonization may be the primary source of environmental heterogeneity and may explain inconsistent results from soil heterogeneity experiments.
Similar content being viewed by others
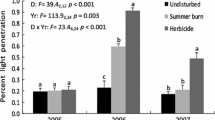
Avoid common mistakes on your manuscript.
Introduction
Spatial heterogeneity is viewed as one of the fundamental drivers of local species diversity patterns (McIntosh 1986; Wright 2002; Lundholm 2009; Stein et al. 2014) and a potential explanation for species coexistence (Reynolds et al. 1997; Hendriks et al. 2015). If species vary in their ability to acquire and utilize different resources or tolerate conditions, a more heterogeneous environment is predicted to support more species (Tilman 1982; Lundholm 2009). Given this intuitive idea, numerous observational studies have examined the link between fine-scale spatial heterogeneity and community diversity (Bowers and McLaughlin 1982; Currie and Paquin 1987; Linder 1991; Collins and Wein 1998; Wilson 2000; Lundholm 2009; Bahram et al. 2015) with most reporting a positive heterogeneity-diversity relationship (HDR) (Lundholm 2009; Stein et al. 2014).
In contrast to observational studies, heterogeneity-diversity experiments yield conflicting results. For example, several experiments report positive HDRs (Fitter 1982; Vivian-Smith 1997; Wilson 2000; Williams and Houseman 2014) while others find no effect (Grime et al. 1987; Collins and Wein 1998; Stevens and Carson 2002; Baer et al. 2004; Wijesinghe et al. 2005; Reynolds et al. 2007), or even a negative effect of heterogeneity on diversity (Gazol et al. 2013). Among the experiments that have reported a positive HDR, there is no single type of manipulation that appears to increase diversity by allowing for increased species sorting in heterogeneous environments. However, experiments that decreased the patch size (grain size) of heterogeneity tended to result in positive HDRs (Tamme et al. 2010). The mismatch between observational and experimental studies is puzzling, but several possible explanations have begun to emerge.
First, the type of manipulation utilized in the experiments cited above may create artefacts that undermine heterogeneity-diversity processes. For example, a common strategy is to use inorganic fertilizer to create patches that vary in nutrient conditions, resulting in plots with different levels of heterogeneity (Jackson and Caldwell 1996; Fransen et al. 2001; Xue et al. 2018b). This approach is conceptually strong because of the clear linkage between heterogeneous soil resource conditions and niche partitioning. However, the patches may have elevated nutrient levels that exceed natural conditions, potentially suppressing plant diversity (Reynolds et al. 2007). Additionally, fertilizer may disrupt the natural interactions between plants and soils (Collins and Wein 1998; Bliss et al. 2002), which would then alter species sorting and niche availability, further impacting HDRs. A few other experiments have recognized the potential complications of inorganic fertilizer and have utilized other means to create soil heterogeneity by manipulating soil depth (Baer et al. 2016) or soil type (Williams and Houseman 2014), yet the results of these studies suggest very modest or perhaps transient effects (unpublished data collected by authors ELK and GRH as a continuation of the experiment conducted in Williams and Houseman 2014).
A second reason that field experiments may yield inconsistent HDR relationships is that plants can create an unaccounted source of heterogeneous spatial structure regardless of the underlying soil heterogeneity (Hinsinger et al. 2005; Schouten and Houseman 2019; López-Angulo et al. 2020). For example, non-uniform seed arrival may lead to an internal (endogenous) source of plant spatial structure that shifts environmental conditions within a patch by altering light and nutrient dynamics (Temperton et al. 2007; Coykendall and Houseman 2014; Roscher et al. 2016) or initiating species-specific plant-soil feedbacks (Xue et al. 2018a). A good example of this phenomenon was reported by Schouten and Houseman (2019) who found that, despite using homogenized soils and homogenous seed addition, experimental plots in tallgrass prairie developed aggregated rather than random or uniform spatial structure. If such endogenously driven spatial structure is common, it may explain why positive HDR patterns are widely reported in observational studies but equivocal in experimental studies that do not account for endogenous plant spatial structure.
For heterogeneity to have a positive impact on diversity, grain size (patch size) must be large enough for organisms to utilize the entirety of a particular patch (Lundholm 2009; Laanisto et al. 2013; Stein et al. 2014). In communities of equal size (spatial extent), plant diversity is expected to increase as patch size decreases, because there are more patch types available. However, smaller patches result in fewer individuals per patch, increasing vulnerability to stochastic mortality and local extinction. This microfragmentation hypothesis has been proposed to explain negative HDRs reported in some studies (Laanisto et al. 2013). Because few experiments incorporate multiple patch sizes, scale dependency may also contribute to the disparity between observational and experimental HDR studies.
To our knowledge, no experiments have examined the potential interactive effects of soil heterogeneity, aggregated seed arrival, and patch size (grain size) on plant community diversity. We present results from a field experiment where we manipulated soil heterogeneity, spatial pattern of initial seed arrival and the spatial scale of individual patches in a fully crossed design. In this experiment, we created either heterogenous or homogenous soils and sowed seeds in either intraspecific patches or uniformly throughout a plot while also changing the patchiness of each type of heterogeneity. After four years of community assembly, we use this experiment to address two questions: 1) How do soil heterogeneity, aggregated seed arrival, and the patchiness of heterogeneity affect total species richness on their own and interactively? 2) Do these effects differ if a species was sown into a plot or if it was established in a plot from the surrounding grasslands or underlying seed bank (i.e. non-sown)? We also examine which sown and non-sown species are driving changes in plant diversity.
Methods
Study site
We established experimental plots in a restored prairie at Wichita State University’s Ninnescah Field Station (Latitude: 37.5392, Longitude: -97.6810, Kansas, USA). The station was under row-crop agriculture until 1984 when it was restored to native grassland and subsequently managed using periodic burning, mowing, and haying. Currently, perennial native grasses, and scattered perennial native forbs and legumes dominate the field. The site averages 782 mm of precipitation annually and has a mean annual temperature of 14.3 °C (Houseman et al. 2016). Soil was made up of sandy loam soils that rarely flood (Soil Survey Staff 2022). No flooding occurred during this experiment.
Field experiment
We selected an area that was approximately 40 × 200 m in a restored grassland on similar soils to a previous soil heterogeneity experiment (Schouten and Houseman 2019; Williams and Houseman 2014). In this area, we established eight blocks that were approximately 12 × 20 m (Fig S1). Each block was comprised of twelve, 4 × 4.8 m plots with at least a one-meter buffer on each side (Fig. 1). We assigned 10 treatment combinations and two control plots in a randomized, complete block design (Fig. 1). Plots consisted of either large-scale patches (40 × 40 cm), small-scale patches (20 × 20 cm), or no patches (only found in control plots; Fig. 1). This meant that while the grain of heterogeneity (patch size) varied between plots, the extent (physical size) of each plot did not vary. Patches contained different soil types creating either homogenous, heterogeneous, or non-manipulated soils (Figs. 1 and 2). Additionally, these patches within a plot were sown with either one of 40 native species (see Table S1; Figs. 1 and 2), all species, or no species, creating aggregated, uniform seed arrival, or no seed arrival (control) respectively (Fig. 1). Consequently, every plot had a different combination of soil heterogeneity, seed arrival, and patch size (Fig. 1). This paper will only address those plots that included soil manipulation.
A map of a hypothetical block of twelve plots. The background color of each plot represents the soil heterogeneity of each plot. Green represents heterogeneous soils (Het), blue represents homogenous soils (Hom), and yellow and grey represent unmanipulated soils (Unmanip). The shade of each color represents the seed arrival within a plot. Darker shades represent aggregated seed arrival while lighter shades represent uniform seed arrival. Finally, the grid inside each plot represents the size of patches within a plot. A grid with large spaces represents plots with large patches, a small grid represents those with small patches, and no grid for plots with unmanipulated soils. All plots that had experimental seed arrival, but had unmanipulated soils had large patches. Within a block, there is one replicate of each treatment combination, except for true controls which had two replicates
Example of soil manipulation and seed addition utilized to create plots with large patches for heterogeneous or homogenous soils and aggregated or uniform seed arrival treatments. Plots with heterogeneous soils had three approximately 15 cm soil strata removed from the soil profile, separated, and then each was mixed separately to create three soil types (soil types A, B, C; green, yellow, and grey patches, respectively). These soil types were then randomly redistributed into 120, 40 × 40 cm patches, creating heterogeneous soils. In plots with homogenous soils, the same soil strata were removed, but all strata (mixed soil type, brown patches) were mixed together and then redistributed into all 120 patches in the plot. After the soil manipulation, seeds from 40 native species were sown into each plot. Either one species was sown per patch to create aggregated seed arrival (numbers 1–40), or all 40 species were sown into every patch (All) to create uniform seed arrival at the plot scale. The manipulations depicted in this figure represent the experimental manipulation for plots with large patches. Plots with small patches received the same soil manipulation and seed arrival treatment; however, instead of 120, 40 × 40 patches, plots were comprised of 480, 20 × 20 cm patches
Soil manipulation
To prepare the soil for manipulation, the field site was treated with herbicide (9% glyphosate), over an area of approximately 40 × 200 m in September 2016 (Fig S1). We cut standing dead vegetation and the underlying litter with a weed whip, which was then raked off the plots. We allowed the site to rest for 7 weeks after the herbicide treatment before the soil manipulation began.
Following the approach used in Williams and Houseman (2014) we created the different soil treatments by excavating three strata from the vertical soil profile of plots designated for soil manipulation (Fig. 2). Each stratum was approximately 15 cm deep. We expected soil strata to vary in soil nutrients, texture, organic matter, and soil organisms (see Schouten and Houseman 2019; Fig. 2). For heterogeneous treatments, we randomly redistributed the strata into patches within a plot (Fig. 2). For plots with homogenous soils, all three strata were evenly mixed and then redistributed into patches. Soil manipulations were conducted on one plot at a time, this allowed for the native soil in each plot to remain constant while maximizing (heterogeneous plots) or minimizing soil patchiness (homogenous plots; Fig. 2).
We used a Skid-Steer, to excavate the soils within a plot. Once removed, we placed the soil from homogenous plots incrementally into a single bin, with each stratum filling one-third of the bin. Next, we mixed the three strata to create a homogenous mixture of all three strata. We placed the soil from heterogeneous plots into separate bins outside of the experimental area, one for each stratum, and then mixed each with a rototiller to control for the mixing effect in homogenous treatments (Fig. 2).
To create soil patches of a known size, a plywood form was placed within the plot. For plots with large patches, the openings were 0.4 × 0.4 m resulting in 120 patches per plot. For the smaller patch size, we inserted dividers into each opening to further subdivide the patch into 0.2 × 0.2 m openings yielding 480 patches per plot. For heterogeneous plots, we randomly assigned each stratum to an opening (patch) and filled it with the appropriate soil type. We filled all patches to approximately the same level. After filling each patch, the form was extracted using a forklift. We used our feet to compress soils as necessary during and after filling. Plots with homogenous soil were filled in the same manner, only using the homogenized soil (Fig. 2). After the completion of the soil manipulation, we placed a polypropylene cloth over the plots to minimize wind disturbance.
Seed distribution
To prepare the site for seed addition and to minimize the influence of competition from the pre-existing seed bank, we allowed the seed bank to germinate, and then emerging plants were killed with glyphosate in May 2017. After the plants senesced, we removed emergent plants and then sowed the seeds of 40 native prairie species into each plot (Table S1). For both uniform and aggregated seeding treatments, we sowed seeds into the patches created during the soil manipulation. This meant that any given patch had a soil type (upper, middle, lower, or mixed strata), initial seed arrival (aggregate or uniform), and size (small or large) (Figs. 1 and 2).
In aggregated plots, we sowed one of forty native tallgrass prairie species (Table S1) per soil patch. In plots with large patches, we sowed each species into 3 randomly selected soil patches, while in plots with small patches, we sowed each species into 12 randomly selected soil patches. To create uniform plots, we sowed seeds from all forty species into each soil patch. In plots with large patches, heterogeneous soils, and aggregated seed arrival, there was only one replicate of each soil type and seed combination (three soil types and forty species make 120 patches); in small-scale aggregated plots with heterogeneous soils, there were four replicates of each soil type and species combination. The forty native species were perennial species that represented a range of functional groups and plant families (Table S1). For each species, we held the number of seeds sown into a plot constant, regardless of treatment.
After seed sowing, a biodegradable germination mat was placed over plots to protect the seeds from wind and water movement and granivores, as well as to promote germination. We watered plots periodically until midway through the first growing season, to prevent seed desiccation. Additionally, following plot establishment and seed sowing, we regularly mowed the vegetation around the perimeter of each plot and in between and around blocks to limit the input of non-sown seeds into our plots. Mowing occurred approximately once a month from May to September each year (2018–2020).
Plot sampling
We recorded percent cover for all species in all plots during August of every year (2018–2020). Within a plot, every plant was identified to species or morphospecies, and then their cover within a plot was measured to the nearest percentage. Morphospecies were used for species that could not be accurately distinguished in the field. In our case, sedges from the Carex and Cyperus genera were placed into one morphospecies, Oxalis spp. were placed into another morphospecies, and low-growing Euphorbia species were placed into a third morphospecies.
Phytometer
To evaluate the extent to which our soil manipulations altered the magnitude of soil heterogeneity, we conducted a phytometer assay. This phytometer was set up to test the potential influence of plant-soil feedbacks for the added species in each of the soils described above. Here, we present a subset of those results as a way to quantify soil differences in the field experiment. Using an area adjacent to the experimental plots (Fig. S1), we created soils representing the upper, middle, and lower soil strata as well as homogenized soil conditions (a combination of the three soil strata). We filled 68 L pots with one of the four soil types and then moved each to a randomly assigned position in an area adjacent to the experimental plots. Seeds from one of our 40 species were then sown into each pot.
Following three growing seasons, a greenhouse assay was conducted to assess the effects of soil heterogeneity and plant conditioning on total plant biomass, one way of measuring plant productivity. The plant species used for the greenhouse assay were selected based on their success in the conditioning phase and their relative abundance in the experimental plots. The species chosen represented the key functional groups found in the field experiment: the graminoids Schizachyrium scoparium ([Michx]. Nash) and Sorghastrum nutans ([L.] Nash), the forbs Helianthus maximilliani (Shrad.) and Ratibida pinnata ([Vent.] Barnhart), and the legume Desmodium canadense ([L.] DC.). We used the soil from each field pot to fill 60 conical-shaped pots with a volume of 655 cm3. Approximately 5–10 seeds of each species were sown into each pot and then thinned to one individual plant. After a growth period of twelve weeks, plants were extracted from the pots and split into roots and shoots. Roots were separated from soil by placing the soil in a 4 mm soil sieve suspended in water. We gently agitated the roots to remove the soil and then patted the roots dry with a paper towel. The root or shoot biomass was placed into labeled envelopes and dried for at least forty-eight hours in an oven at 60 °C. Aboveground and belowground biomass were weighed and recorded.
Statistical analysis
For the field experiment, we used R 4.1.2 to test for treatment effects on species richness at the plot scales using a 3-way Analysis of Deviance fitted to a generalized linear mixed-effect model (GLMM) for a randomized complete block design (Bates et al. 2015; Fox and Weisberg 2019; R Core Team 2022). GLMM was used because it allowed us to test both random and fixed effects while also accounting for non-normal distributions. For analyses of total and non-sown species richness, the Poisson distribution with an identity link function was used, while a Poisson distribution with a log link function was used for sown species richness. The GLMMs were then used as the basis for an Analysis of Deviance – Wald Chi-square Test using the ‘Anova’ function from the car package (Fox and Weisberg 2019). Pairwise results for any significant interactions were calculated using the emmeans package in R (Lenth 2020). We also used GLMM as a post hoc test to determine how non-sown species richness varied with the abundance of the species initially sown into aggregate patches. The same GLMM workflow was used to analyze phytometer data, only using the Gamma distribution with an inverse link function. Indicator Species Analyses (ISA) were used as a post hoc test to identify which species were driving the differences between our treatments. (Caceres and Legendre 2009). We specifically focused on treatments that appeared to be different based on our Analysis of Deviance.
Results
Total species richness
Initially, we examined differences in species richness, evenness, and Shannon-diversity between treatments and across time. However, species evenness did not significantly vary between treatments. As a result, trends in Shannon-diversity closely followed trends in richness. Additionally, in years one and two, plant communities were too dynamic/unstable to be able to adequately quantify how they varied between treatments, and we wanted to be reasonably certain that perennial plants had fully established, allowing for any-time dependent effects of heterogeneity on diversity to emerge. For simplicity, we elected to only present species richness results from year four. Total species richness at the plot scale varied among treatments, but this response was dependent on a marginally significant two-way interaction between initial seed arrival and patch size (p = 0.071, Tables 1a and 2a). To understand this interaction, we first examined the results from small- and large-scale treatments separately. For plots with small patches, aggregated seed arrival led to higher species richness than uniform seed arrival, regardless of soil heterogeneity (p = 0.005, Tables 1a and 2a, Fig. 3a). This pattern was also present in plots with large patches (p < 0.001, Fig. 3a). Specifically, the difference in seed arrival patterns was marginally more pronounced in plots composed of large patches compared to plots with small patches (p = 0.073, Fig. 3a, Table 2a).
The average (a) total richness, (b) sown species richness, and (c) non-sown species richness of each treatment combination after four growing seasons. Each panel is split into two sub-panels with plots with small patches on the left and large patches on the right. Within a sub-panel, the x-axis represents initial seed arrival (uniform and aggregated), and each tick mark is divided into our two soil types (heterogeneous and homogeneous). The y-axis represents species richness for a given subset of species (total, sown, and non-sown). Error bars represent 95% confidence intervals. Compact letter displays indicate differences between treatments as indicated by the Analysis of Deviance and subsequent pairwise comparisons. Total species richness was marginally impacted by the interaction of seed arrival and patch size (Χ2 = 3.25, p = 0.071). Sown species richness was only marginally impacted by seed arrival (Χ2 = 3.77, p = 0.052). Non-sown species richness was significantly influenced by the three-way interaction between soil heterogeneity, seed arrival, and patch size (Χ2 = 5.46, p = 0.019)
Sown vs. non-sown species richness
Because a total of 60% of the species observed across the entire experiment were non-sown, we also analyzed the responses of sown and non-sown species separately to determine if the treatment responses were consistent across the two groups. For the sown species, seed aggregation was the only factor that marginally influenced species richness (p = 0.052, Table 1b). Specifically, sown species richness was higher in plots with aggregated than uniform seed arrival regardless of soil heterogeneity or patch size (Fig. 3b).
In general, plots with large patches had higher non-sown species richness than those with small patches (p = 0.004, Table 1c). Additionally, aggregated seed arrival resulted in higher non-sown richness than uniform seed arrival regardless of the underlying soil (p < 0.001, Table 1c, Fig. 3c). For plots with heterogenous soils, this difference was more pronounced in plots with large patches (p < 0.001; Table 2b, Fig. 3c), this difference was not present for plots with homogenous soils (Table 2b). Soil heterogeneity only marginally affected non-sown species richness (p = 0.080, Table 2b). Specifically, plots with uniform seed arrival and small patches had higher non-sown richness when the underlying soils were heterogeneous instead of homogenous (p = 0.080, Table 2b). There were no other treatment combinations that differed by soil heterogeneity (Table 2b). When taking all experimental factors into account, the size of patches within plots only influenced non-sown species richness when soils were heterogeneous and seed arrival was aggregated (Table 2b). Under these conditions, plots with large patches had higher non-sown species richness than those with small patches (p = 0.004, Table 1c). Patch size did not influence non-sown species richness when seed arrival was uniform (Table 2c).
Indicator species analysis
We used Indicator Species Analysis (ISA) to determine which species were driving the differences we observed in total, sown, and non-sown species richness. Specifically, we used ISA to make single treatment comparisons (i.e. to compare aggregated and uniform seed arrival) for treatment combinations that significantly impacted total, sown, or non-sown species richness (Table 3). We found that eight non-sown species were indicators in multiple treatment combinations (Table 3; Ambrosia artemisiifolia (L.), Cirsium altissimum ([L.] Hill), Lactuca ludoviciana ([Nutt.] Riddell), Rumex crispus (L.), Conyza canadensis ([L.] Cronquist), Populus deltoides (W. Bartram ex Marshall), Agrostis hyemalis ([Walter] Britton, Sterns & Poggenb.), and the Carex and Cyperus spp. morphospecies). All eight non-sown indicator species were species that are generally considered to be early successional or favor recently disturbed areas. We also found that 24 unique species were more likely to be found in certain treatment combinations, though none were indicators for treatment combinations that involved all three experimental factors (Table 3). Regardless of species origin (sown or non-sown), species were generally indicators of treatment combinations that included aggregated seed arrival, large patches, or both (Table 3).
Phytometer
To determine whether we created soil patches with ecologically important differences in growing conditions, we measured the total plant biomass of five plant species grown in our three heterogeneous soils and our one homogenous soil in a greenhouse experiment (Table S2a). We found significant differences among the three soils that comprised patches in the heterogeneous plots (p < 0.001, Table S2a). Total plant biomass was highest on soils from the upper soil stratum. On average, biomass was decreased by 34.1 percent on soils from the middle stratum and was decreased by 50.6 percent on soils from the lower stratum (Table S2b; Figs. S2-S7). Additionally, when grown in mixed soils, total biomass decreased by 27.5 percent compared to those grown in the upper soil stratum (Table S2b).
Discussion
Our field experiment revealed that aggregated seed arrival had much stronger effects on plot species richness than soil heterogeneity, and these differences occurred regardless of the patch size examined. The seed arrival treatments had similar effects on both sown species and non-sown species which arrived through seed dispersal or seedbanks. Soil heterogeneity did have weak effects, but only for the non-sown species. The phytometer analysis revealed clear differences in the three soils that comprised heterogeneous soil plots. However, these realistic levels of fine-scale soil heterogeneity did not strongly contribute to differences in plot species richness, while the spatial structuring of seed arrival clearly increased it.
Seed arrival
Plots that were initially established with aggregated seed arrival had higher species richness than those established by uniform seed arrival. This trend held true for total, sown, and non-sown species richness (Fig. 3). Our results are consistent with other work that has demonstrated that spatial heterogeneity created by sowing seeds in intraspecifically aggregated clusters increases diversity (Houseman 2014; Xue et al. 2018b). For both sown and non-sown species, increased richness in plots with aggregated compared with uniform seed arrival likely reflects reduced interspecific competition relative to intraspecific competition (Moore et al. 2001; Houseman 2014; Velázquez et al. 2014; Ghazian et al. 2021). If true, this supports the idea that aggregated seed arrival can alter community assembly and allow for the increased establishment of volunteer species.
While our sown species were purposefully introduced, the source of our non-sown species is less clear. These species may have originated from the underlying seedbank or dispersal from areas adjacent to the plot or the seedbank after the initial experimental seed arrival. Additionally, some sown species occurred in the surrounding community and may have also been present in the underlying seedbank (no more than 10 sown species occurred in the surrounding community; personal observation). Although we cannot quantify the relative contributions from dispersal versus the seedbank for both sown and non-sown species, we took measures during plot setup to reduce the effects of seedbank input by applying a foliar herbicide to eradicate species emerging from the seedbank prior to the addition of the sown species. When adding the sown species to plots we used seed addition rates that were reflective of moderate prairie production and were likely to ensure sufficient germination (see Houseman 2014). Meaning that any sown species observed in a plot likely came from the initial experimental manipulation and not seedbank germination or dispersal. We also took measures during the experiment to limit seed input from areas adjacent to the plot by mowing the areas in between and around the plots. However, species were likely to disperse across the relatively small areas around the plots. The combination of the seedbank and natural levels of dispersal in this field contributed to total richness in important ways, depending on the spatial structure of the sown species, patch size, and soil heterogeneity.
Why was non-sown species richness higher in aggregated than uniformly sown plots? One possible explanation is that spatial aggregation coupled with plant-soil feedbacks generates environmental heterogeneity that enhances opportunities for other species, thus increasing species richness in the plots. For example, species such as Lespedeza capitata (Michx.) and Baptisia australis ([L.] R. Br.) can facilitate the growth of conspecifics through plant-soil feedbacks and the enhancement of nitrogen-fixing mutualists (Temperton et al. 2007; Coykendall and Houseman 2014; Roscher et al. 2016). While the initial intraspecific aggregation in our experiment was artificially created, it has been shown that species can naturally aggregate through endogenous processes over time even under experimentally homogenized conditions (Schouten and Houseman 2019). Variation in the direction and magnitude of plant-soil feedbacks likely alters both abiotic and biotic environments. Consequently, the plots with aggregated spatial arrangement of sown species may enhance environmental heterogeneity.
A second possible explanation for the higher colonization of non-sown species in aggregated sowing plots is that some sown species did not monopolize patches despite an overwhelming seed advantage. Although most of the sown species established somewhere in the experiment (38 out of 40), these species varied in their ability to either establish, persist, or grow during the first few years of the experiment. This within-plot variability was less likely in uniform plots where all 40 species were sown into each patch in contrast to aggregated plots where a single species was sown into each patch. Consequently, the establishment of fast-growing species was much more likely in patches within uniform plots (Wardle 1999). The increased likelihood of poor establishment or growth in aggregated plots likely creates more patches with various dominant competitors and abiotic conditions present, creating more opportunities for non-sown species to establish (Melbourne et al. 2007; Velázquez et al. 2014; Ghazian et al. 2021). This view is at least partially supported by the negative correlation between non-sown species richness and the cover of sown species in aggregated patches (Fig. S8). Interestingly, seven of the eight non-sown indicator species established more often and in higher abundance in plots with aggregated seed arrival (Table 3). These seven non-sown indicator species are all species that establish well following a disturbance, and as such, commonly occur during the early stages of community assembly when our study took place (Haddock et al. 2015). The consistent establishment of the same seven non-sown indicator species suggests that these same species are responding to low abundances in certain sown species and thus establishing in relatively low-competition patches. If this interpretation is correct, aggregated seed arrival may increase environmental heterogeneity either independently or in conjunction with the underlying soil heterogeneity.
Previous studies have similarly suggested that aggregated seed arrival may either increase environmental heterogeneity in tandem or independently of the underlying soil heterogeneity. For example, evidence from a greenhouse experiment suggests that different sources of spatial structure, such as soil heterogeneity and plant-mediated spatial structure, can have independent and interactive impacts on plant communities, and that some plant species may be insensitive to different sources of spatial structure (Xue et al. 2018b). While the results presented in Xue et al. (2018b) are from a relatively simple plant community in a greenhouse, similar processes may be happening in larger field experiments. Namely, it seems possible that plant species with high plasticity, or the ability to adapt to variable and changing environmental conditions, may also be relatively insensitive to changes in environmental heterogeneity. Further experiments or modeling are needed to confirm this idea.
Soil heterogeneity
Soil heterogeneity on its own did not significantly influence species richness. However, when seeds were sown uniformly, plots with homogenous soils had higher total richness than those with heterogeneous soils. This result contradicts many of the positive HDRs reported in Lundholm (2009). Likewise, our results also contradict the positive effect of soil heterogeneity on plant diversity reported in Williams and Houseman (2014), which occurred in a similar system (less than 300 m east of our experiment). One possible reason for the latter difference is that differences in soil strata may have resulted in smaller differences between soil types than Williams and Houseman (2014). However, our phytometer revealed clear differences in plant biomass among the three soil types that were used in the heterogeneous soil treatment, indicating that differences between soil types were biologically meaningful.
While soil heterogeneity may not have influenced species richness as strongly as we anticipated, it did affect the community in other ways. For example, plant growth in the phytometer varied based on the soil type they were grown in (Fig. S2-S7, Table S2). Despite clear differences based on soil type, these differences did not persist at the plot-scale. This aligns with the idea that native perennial prairie species and communities, particularly those that are clonal, are robust to variation in the soil while weedier, non-sown species are more sensitive to soil variation (Reynolds et al. 2007). Certainly, large differences in soil types would be expected to yield high species sorting, but it is unlikely that such soil variation would occur at fine scales. In our system, prairie species are adapted to strong variation in temperature and precipitation which means that they may not be sensitive to our relatively modest soil type differences, explaining why there is little species sorting among the soil types in our experiment.
Patch/grain size
The effects of spatial aggregation on our plant communities were also dependent on the size of patches comprising our plots (Fig. 3, Table 1). Even though all plots covered the same area, all species, regardless of origin, had lower establishment in the plots with smaller patches as predicted by the microfragmentation hypothesis (Laanisto et al. 2013). This may have occurred because fewer individuals can establish in a patch, thus increasing stochastic local extinction. Secondly, small patches have a greater edge to interior ratio, potentially increasing the negative influence of neighboring patches on species establishment. Interestingly, we found a lesser effect of patch size on the establishment of sown species, possibly because such microfragmentation effects take longer for well-established species.
Conclusions
Our experiment provides empirical evidence that fine-scale soil heterogeneity has weak effects on prairie plant species richness, but that non-uniform seed arrival can have stronger effects. It appears that spatial patterns in seed arrival can generate spatially-structured environmental heterogeneity, perhaps by creating greater variability in resources, plant-soil feedbacks, or the competitive milieu. These results suggest that environmental heterogeneity is primarily generated endogenously following plant colonization. Such processes would be consistent with positive HDRs often reported in observational studies, and may explain why manipulative soil heterogeneity experiments generally do not report strong HDR patterns.
Data availability
The datasets analyzed during the current study are available in the figshare repository, Plot-Level Data: https://doi.org/10.6084/m9.figshare.14893398.v1, Phytometer Data: https://doi.org/10.6084/m9.figshare.21365658.v3 R code for data analyses associated with the current study are available on GitHub: https://github.com/elkjaer1745/SoilHet2_4yrs_CommunityAssembly.
References
Baer SG, Blair JM, Collins SL (2016) Environmental heterogeneity has a weak effect on diversity during community assembly in tallgrass prairie. Ecol Monogr 86:94–106. https://doi.org/10.1890/15-0888.1
Baer SG, Blair JM, Collins SL, Knapp AK (2004) Plant community responses to resource availability and heterogeneity during restoration. Oecologia 139:617–629. https://doi.org/10.1007/s00442-004-1541-3
Bahram M, Peay KG, Tedersoo L (2015) Local-scale biogeography and spatiotemporal variability in communities of mycorrhizal fungi. New Phytol 205:1454–1463. https://doi.org/10.1111/nph.13206
Bliss KM, Jones RH, Mitchell RJ, Mou PP (2002) Are competitive interactions influenced by spatial nutrient heterogeneity and root foraging behavior? New Phytol 154:409–417. https://doi.org/10.1046/j.1469-8137.2002.00389.x
Bowers JE, McLaughlin SP (1982) Plant species diversity in Arizona. Madroño 29:227–233
Collins B, Wein G (1998) Soil resource heterogeneity effects on early succession. Oikos 82:238–245. https://doi.org/10.2307/3546963
Coykendall KE, Houseman GR (2014) Lespedeza cuneata invasion alters soils facilitating its own growth. Biol Invasions 16:1735–1742. https://doi.org/10.1007/s10530-013-0623-8
Currie DJ, Paquin V (1987) Large-scale biogeographical patterns of species richness of trees. Nature 329:326. https://doi.org/10.1038/329326a0
Caceres M, Legendre P (2009) Associations between species and groups of sites: indices and statistical inference. Ecology 90:3566–3574. https://doi.org/10.1890/08-18231
Fitter AH (1982) Influence of Soil Heterogeneity on the Coexistence of Grassland Species. J Ecol 70:139–148. https://doi.org/10.2307/2259869
Fransen B, de Kroon H, Berendse F (2001) Soil Nutrient Heterogeneity Alters Competition Between Two Perennial Grass Species. Ecology 82:2534–2546. https://doi.org/10.1890/0012-9658(2001)082[2534:SNHACB]2.0.CO;2
Gazol A, Tamme R, Price JN et al (2013) A negative heterogeneity-diversity relationship found in experimental grassland communities. Oecologia 173:545–555. https://doi.org/10.1007/s00442-013-2623-x
Ghazian N, Braun J, Owen M et al (2021) Seed aggregation tips the scale in plant competition. Commun Ecol 22:403–412. https://doi.org/10.1007/s42974-021-00064-5
Grime JP, Mackey JML, Hillier SH, Read DJ (1987) Floristic diversity in a model system using experimental microcosms. Nature 328:420. https://doi.org/10.1038/328420a0
Haddock MJ, Freeman CC, Bare J (2015) Kansas wildflowers and weeds. University Press of Kansas, Lawrence
Hendriks M, Ravenek JM, Smit-Tiekstra AE et al (2015) Spatial heterogeneity of plant-soil feedback affects root interactions and interspecific competition. New Phytol 207:830–840. https://doi.org/10.1111/nph.13394
Hinsinger P, Gobran GR, Gregory PJ, Wenzel WW (2005) Rhizosphere geometry and heterogeneity arising from root-mediated physical and chemical processes. New Phytol 168:293–303. https://doi.org/10.1111/j.1469-8137.2005.01512.x
Houseman GR (2014) Aggregated seed arrival alters plant diversity in grassland communities. J Plant Ecol 7:51–58. https://doi.org/10.1093/jpe/rtt044
Houseman GR, Kraushar MS, Rogers CM (2016) The Wichita State University Biological Field Station: Bringing Breadth to Research Along the Precipitation Gradient in Kansas. Trans Kans Acad Sci 119:27–32. https://doi.org/10.1660/062.119.0106
Jackson RB, Caldwell MM (1996) Integrating resource heterogeneity and plant plasticity: Modelling nitrate and phosphate uptake in a patchy soil environment. J Ecol 84:891–903. https://doi.org/10.2307/2960560
Laanisto L, Tamme R, Hiiesalu I et al (2013) Microfragmentation concept explains non-positive environmental heterogeneity-diversity relationships. Oecologia 171:217–226. https://doi.org/10.1007/s00442-012-2398-5
Linder HP (1991) Environmental correlates of patterns of species richness in the south-western Cape Province of South Africa. J Biogeogr 18:509–518. https://doi.org/10.2307/2845687
López-Angulo J, de la Cruz M, Chacón-Labella J et al (2020) The role of root community attributes in predicting soil fungal and bacterial community patterns. New Phytol 228:1070–1082. https://doi.org/10.1111/nph.16754
Lundholm JT (2009) Plant species diversity and environmental heterogeneity: Spatial scale and competing hypotheses. J Veg Sci 20:377–391. https://doi.org/10.1111/j.1654-1103.2009.05577.x
McIntosh RP (1986) The background of ecology: concept and theory. Cambridge University Press, Cambridge
Melbourne BA, Cornell HV, Davies KF et al (2007) Invasion in a heterogeneous world: resistance, coexistence or hostile takeover? Ecol Lett 10:77–94. https://doi.org/10.1111/j.1461-0248.2006.00987.x
Moore JL, Mouquet N, Lawton JH, Loreau M (2001) Coexistence, saturation and invasion resistance in simulated plant assemblages. Oikos 94:303–314. https://doi.org/10.1034/j.1600-0706.2001.940211.x
Reynolds HL, Hungate BA, Chapin FS, DAntonio CM, (1997) Soil heterogeneity and plant competition in an annual grassland. Ecology 78:2076–2090. https://doi.org/10.1890/0012-9658(1997)078[2076:SHAPCI]2.0.CO;2
Reynolds HL, Mittelbach GG, Darcy-Hall TL et al (2007) No effect of varying soil resource heterogeneity on plant species richness in a low fertility grassland. J Ecol 95:723–733. https://doi.org/10.1111/j.1365-2745.2007.01252.x
Roscher C, Schmid B, Kolle O, Schulze E-D (2016) Complementarity among four highly productive grassland species depends on resource availability. Oecologia 181:571–582. https://doi.org/10.1007/s00442-016-3587-4
Schouten OS, Houseman GR (2019) Effect of soil heterogeneity and endogenous processes on plant spatial structure. Ecology 100:e02837. https://doi.org/10.1002/ecy.2837
Soil Survey Staff (2022) Natural Resources Conservation Service, United States Department of Agriculture, Web Soil Survey. Available online. https://websoilsurvey.nrcs.usda.gov/app/. Accessed 15 Oct 2022
Stein A, Gerstner K, Kreft H (2014) Environmental heterogeneity as a universal driver of species richness across taxa, biomes and spatial scales. Ecol Lett 17:866–880. https://doi.org/10.1111/ele.12277
Stevens MHH, Carson WP (2002) Resource quantity, not resource heterogeneity, maintains plant diversity. Ecol Lett 5:420–426. https://doi.org/10.1046/j.1461-0248.2002.00333.x
Tamme R, Hiiesalu I, Laanisto L et al (2010) Environmental heterogeneity, species diversity and co-existence at different spatial scales. J Veg Sci 21:796–801. https://doi.org/10.1111/j.1654-1103.2010.01185.x
Temperton VM, Mwangi PN, Scherer-Lorenzen M et al (2007) Positive interactions between nitrogen-fixing legumes and four different neighbouring species in a biodiversity experiment. Oecologia 151:190–205. https://doi.org/10.1007/s00442-006-0576-z
Tilman D (1982) Resource competition and community structure. Monogr Popul Biol 17:1–296
Velázquez J, Garrahan JP, Eichhorn MP (2014) Spatial Complementarity and the Coexistence of Species. PLoS ONE 9:e114979. https://doi.org/10.1371/journal.pone.0114979
Vivian-Smith G (1997) Microtopographic Heterogeneity and Floristic Diversity in Experimental Wetland Communities. J Ecol 85:71–82. https://doi.org/10.2307/2960628
Wardle DA (1999) Is “Sampling Effect” a Problem for Experiments Investigating Biodiversity-Ecosystem Function Relationships? Oikos 87:403–407. https://doi.org/10.2307/3546757
Wijesinghe DK, John EA, Hutchings MJ (2005) Does pattern of soil resource heterogeneity determine plant community structure? An experimental investigation. J Ecol 93:99–112. https://doi.org/10.1111/j.0022-0477.2004.00934.x
Williams BM, Houseman GR (2014) Experimental evidence that soil heterogeneity enhances plant diversity during community assembly. J Plant Ecol 7:461–469. https://doi.org/10.1093/jpe/rtt056
Wilson SD (2000) Heterogeneity, diversity and scale in plant communitites. In: The ecological consequences of environmental heterogeneity. Blackwell Scientific, Oxford, pp 53–69
Wright SJ (2002) Plant diversity in tropical forests: A review of mechanisms of species coexistence. Oecologia 130:1–14. https://doi.org/10.1007/s004420100809
Xue W, Berendse F, Bezemer TM (2018a) Spatial heterogeneity in plant–soil feedbacks alters competitive interactions between two grassland plant species. Funct Ecol 32:2085–2094. https://doi.org/10.1111/1365-2435.13124
Xue W, Huang L, Yu F-H, Bezemer TM (2018b) Intraspecific aggregation and soil heterogeneity: competitive interactions of two clonal plants with contrasting spatial architecture. Plant Soil 425:231–240. https://doi.org/10.1007/s11104-018-3578-9
Acknowledgements
We thank numerous field technicians who helped collect patch-level data each summer. We thank Ryan Shellhammer for leading data collection in the absence of ELK. This work was supported by the National Science Foundation award 1456839
Author information
Authors and Affiliations
Contributions
ELK collected the field data, prepared, and analyzed all data. KNL and ELK collected phytometer data. ELK also led the writing of the manuscript. GRH and ELK conceived the field experiment. KNL and GRH conceived the phytometer experiment. GRH, BLF, LL, and AJG secured the funding for the project. All authors made significant contributions to drafts of the manuscript and approved it for publication.
Corresponding author
Ethics declarations
Competing interests
The authors declare no competing interests.
Additional information
Responsible Editor: Amandine Erktan.
Publisher's Note
Springer Nature remains neutral with regard to jurisdictional claims in published maps and institutional affiliations.
Supplementary Information
Below is the link to the electronic supplementary material.
Rights and permissions
Open Access This article is licensed under a Creative Commons Attribution 4.0 International License, which permits use, sharing, adaptation, distribution and reproduction in any medium or format, as long as you give appropriate credit to the original author(s) and the source, provide a link to the Creative Commons licence, and indicate if changes were made. The images or other third party material in this article are included in the article's Creative Commons licence, unless indicated otherwise in a credit line to the material. If material is not included in the article's Creative Commons licence and your intended use is not permitted by statutory regulation or exceeds the permitted use, you will need to obtain permission directly from the copyright holder. To view a copy of this licence, visit http://creativecommons.org/licenses/by/4.0/.
About this article
Cite this article
Kjaer, E.L., Houseman, G.R., Luu, K.N. et al. Spatial pattern of seed arrival has a greater effect on plant diversity than does soil heterogeneity in a grassland ecosystem. Plant Soil (2024). https://doi.org/10.1007/s11104-024-06565-2
Received:
Accepted:
Published:
DOI: https://doi.org/10.1007/s11104-024-06565-2